HHS Human Host Kimberly
VerifiedAdded on 2022/08/17
|13
|8747
|13
AI Summary
Contribute Materials
Your contribution can guide someone’s learning journey. Share your
documents today.
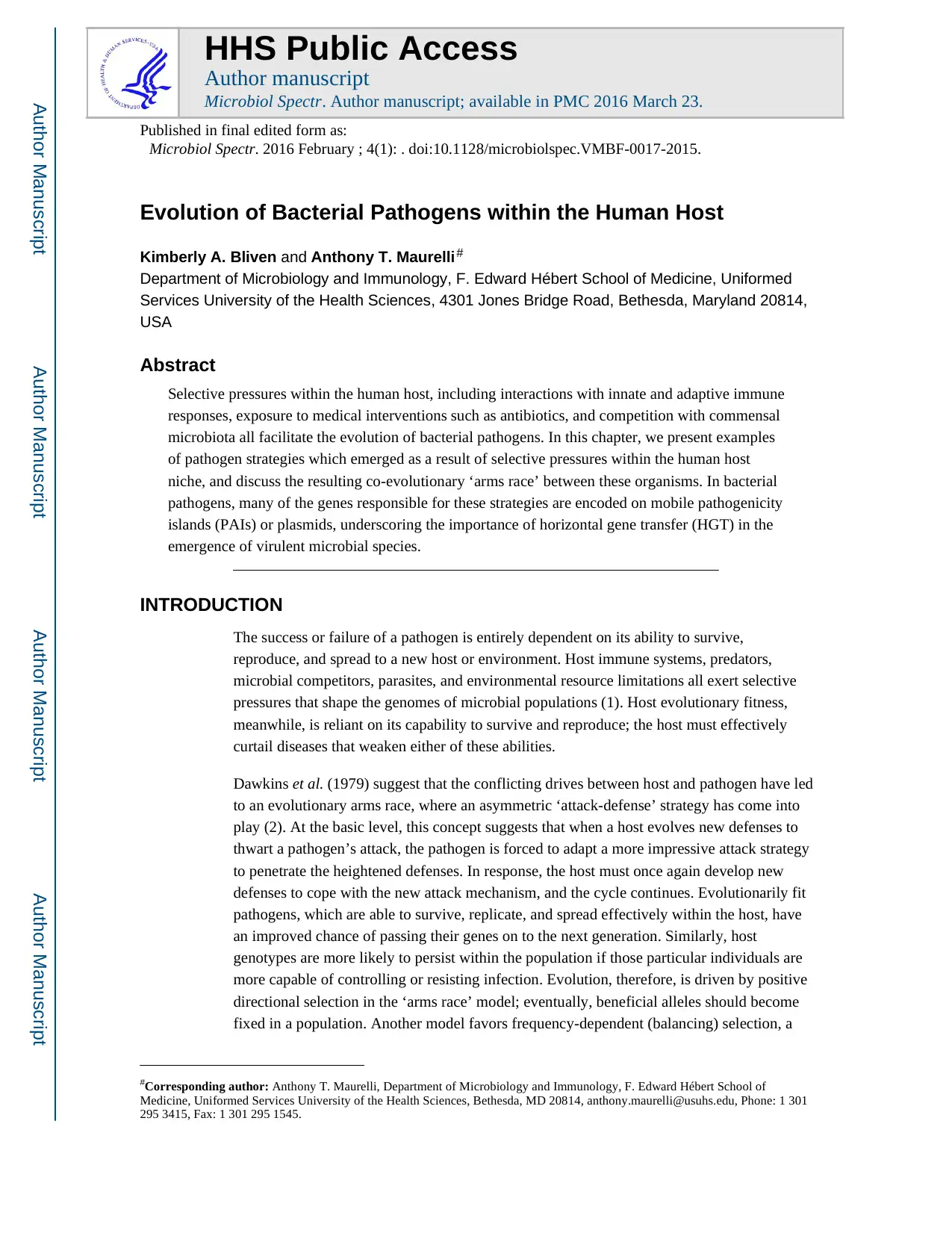
Evolution of Bacterial Pathogens within the Human Host
Kimberly A. Bliven and Anthony T. Maurelli#
Department of Microbiology and Immunology, F. Edward Hébert School of Medicine, Uniformed
Services University of the Health Sciences, 4301 Jones Bridge Road, Bethesda, Maryland 20814,
USA
Abstract
Selective pressures within the human host, including interactions with innate and adaptive immune
responses, exposure to medical interventions such as antibiotics, and competition with commensal
microbiota all facilitate the evolution of bacterial pathogens. In this chapter, we present examples
of pathogen strategies which emerged as a result of selective pressures within the human host
niche, and discuss the resulting co-evolutionary ‘arms race’ between these organisms. In bacterial
pathogens, many of the genes responsible for these strategies are encoded on mobile pathogenicity
islands (PAIs) or plasmids, underscoring the importance of horizontal gene transfer (HGT) in the
emergence of virulent microbial species.
INTRODUCTION
The success or failure of a pathogen is entirely dependent on its ability to survive,
reproduce, and spread to a new host or environment. Host immune systems, predators,
microbial competitors, parasites, and environmental resource limitations all exert selective
pressures that shape the genomes of microbial populations (1). Host evolutionary fitness,
meanwhile, is reliant on its capability to survive and reproduce; the host must effectively
curtail diseases that weaken either of these abilities.
Dawkins et al. (1979) suggest that the conflicting drives between host and pathogen have led
to an evolutionary arms race, where an asymmetric ‘attack-defense’ strategy has come into
play (2). At the basic level, this concept suggests that when a host evolves new defenses to
thwart a pathogen’s attack, the pathogen is forced to adapt a more impressive attack strategy
to penetrate the heightened defenses. In response, the host must once again develop new
defenses to cope with the new attack mechanism, and the cycle continues. Evolutionarily fit
pathogens, which are able to survive, replicate, and spread effectively within the host, have
an improved chance of passing their genes on to the next generation. Similarly, host
genotypes are more likely to persist within the population if those particular individuals are
more capable of controlling or resisting infection. Evolution, therefore, is driven by positive
directional selection in the ‘arms race’ model; eventually, beneficial alleles should become
fixed in a population. Another model favors frequency-dependent (balancing) selection, a
#Corresponding author: Anthony T. Maurelli, Department of Microbiology and Immunology, F. Edward Hébert School of
Medicine, Uniformed Services University of the Health Sciences, Bethesda, MD 20814, anthony.maurelli@usuhs.edu, Phone: 1 301
295 3415, Fax: 1 301 295 1545.
HHS Public Access
Author manuscript
Microbiol Spectr. Author manuscript; available in PMC 2016 March 23.
Published in final edited form as:
Microbiol Spectr. 2016 February ; 4(1): . doi:10.1128/microbiolspec.VMBF-0017-2015.
Author Manuscript Author Manuscript Author Manuscript Author Manuscript
Kimberly A. Bliven and Anthony T. Maurelli#
Department of Microbiology and Immunology, F. Edward Hébert School of Medicine, Uniformed
Services University of the Health Sciences, 4301 Jones Bridge Road, Bethesda, Maryland 20814,
USA
Abstract
Selective pressures within the human host, including interactions with innate and adaptive immune
responses, exposure to medical interventions such as antibiotics, and competition with commensal
microbiota all facilitate the evolution of bacterial pathogens. In this chapter, we present examples
of pathogen strategies which emerged as a result of selective pressures within the human host
niche, and discuss the resulting co-evolutionary ‘arms race’ between these organisms. In bacterial
pathogens, many of the genes responsible for these strategies are encoded on mobile pathogenicity
islands (PAIs) or plasmids, underscoring the importance of horizontal gene transfer (HGT) in the
emergence of virulent microbial species.
INTRODUCTION
The success or failure of a pathogen is entirely dependent on its ability to survive,
reproduce, and spread to a new host or environment. Host immune systems, predators,
microbial competitors, parasites, and environmental resource limitations all exert selective
pressures that shape the genomes of microbial populations (1). Host evolutionary fitness,
meanwhile, is reliant on its capability to survive and reproduce; the host must effectively
curtail diseases that weaken either of these abilities.
Dawkins et al. (1979) suggest that the conflicting drives between host and pathogen have led
to an evolutionary arms race, where an asymmetric ‘attack-defense’ strategy has come into
play (2). At the basic level, this concept suggests that when a host evolves new defenses to
thwart a pathogen’s attack, the pathogen is forced to adapt a more impressive attack strategy
to penetrate the heightened defenses. In response, the host must once again develop new
defenses to cope with the new attack mechanism, and the cycle continues. Evolutionarily fit
pathogens, which are able to survive, replicate, and spread effectively within the host, have
an improved chance of passing their genes on to the next generation. Similarly, host
genotypes are more likely to persist within the population if those particular individuals are
more capable of controlling or resisting infection. Evolution, therefore, is driven by positive
directional selection in the ‘arms race’ model; eventually, beneficial alleles should become
fixed in a population. Another model favors frequency-dependent (balancing) selection, a
#Corresponding author: Anthony T. Maurelli, Department of Microbiology and Immunology, F. Edward Hébert School of
Medicine, Uniformed Services University of the Health Sciences, Bethesda, MD 20814, anthony.maurelli@usuhs.edu, Phone: 1 301
295 3415, Fax: 1 301 295 1545.
HHS Public Access
Author manuscript
Microbiol Spectr. Author manuscript; available in PMC 2016 March 23.
Published in final edited form as:
Microbiol Spectr. 2016 February ; 4(1): . doi:10.1128/microbiolspec.VMBF-0017-2015.
Author Manuscript Author Manuscript Author Manuscript Author Manuscript
Secure Best Marks with AI Grader
Need help grading? Try our AI Grader for instant feedback on your assignments.
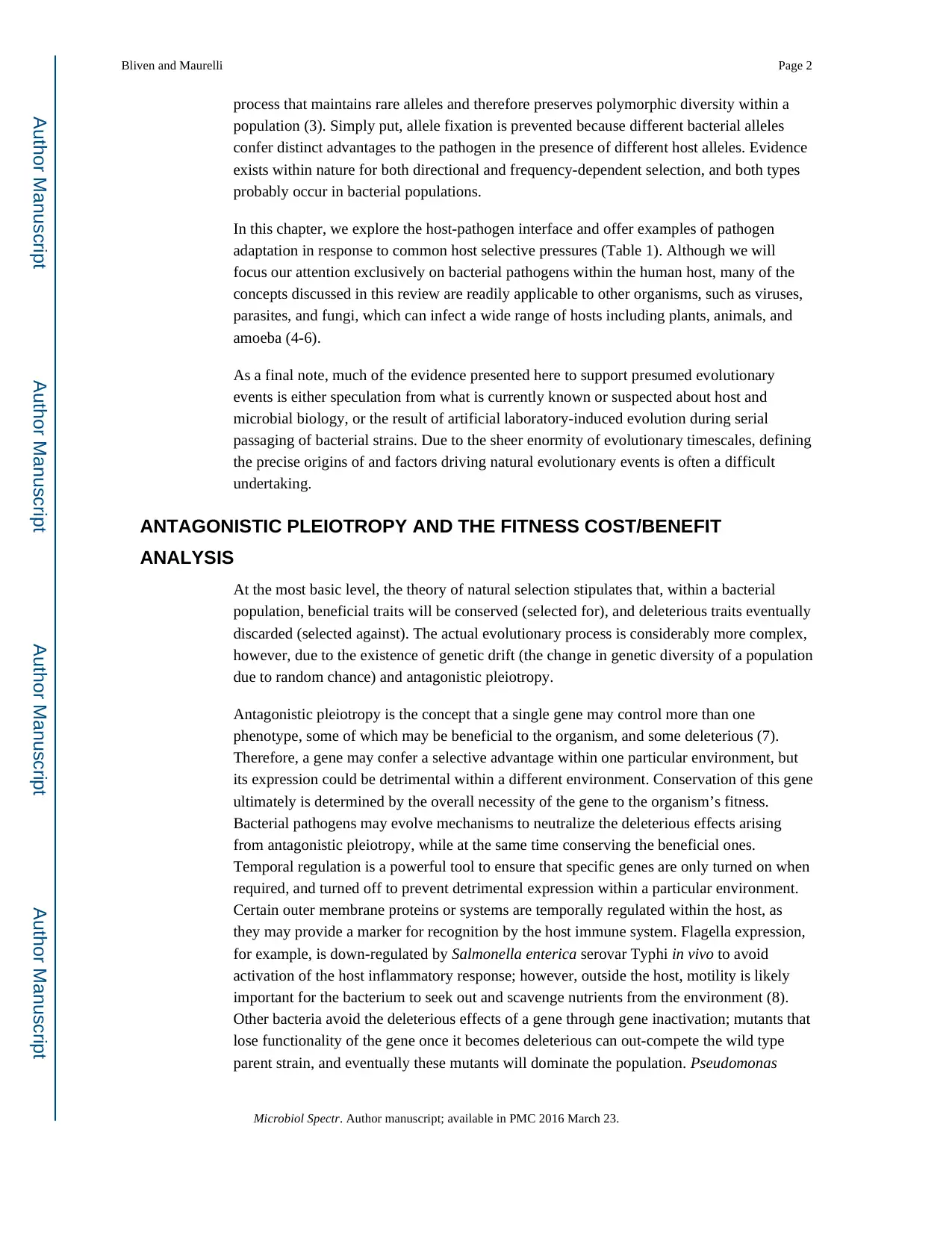
process that maintains rare alleles and therefore preserves polymorphic diversity within a
population (3). Simply put, allele fixation is prevented because different bacterial alleles
confer distinct advantages to the pathogen in the presence of different host alleles. Evidence
exists within nature for both directional and frequency-dependent selection, and both types
probably occur in bacterial populations.
In this chapter, we explore the host-pathogen interface and offer examples of pathogen
adaptation in response to common host selective pressures (Table 1). Although we will
focus our attention exclusively on bacterial pathogens within the human host, many of the
concepts discussed in this review are readily applicable to other organisms, such as viruses,
parasites, and fungi, which can infect a wide range of hosts including plants, animals, and
amoeba (4-6).
As a final note, much of the evidence presented here to support presumed evolutionary
events is either speculation from what is currently known or suspected about host and
microbial biology, or the result of artificial laboratory-induced evolution during serial
passaging of bacterial strains. Due to the sheer enormity of evolutionary timescales, defining
the precise origins of and factors driving natural evolutionary events is often a difficult
undertaking.
ANTAGONISTIC PLEIOTROPY AND THE FITNESS COST/BENEFIT
ANALYSIS
At the most basic level, the theory of natural selection stipulates that, within a bacterial
population, beneficial traits will be conserved (selected for), and deleterious traits eventually
discarded (selected against). The actual evolutionary process is considerably more complex,
however, due to the existence of genetic drift (the change in genetic diversity of a population
due to random chance) and antagonistic pleiotropy.
Antagonistic pleiotropy is the concept that a single gene may control more than one
phenotype, some of which may be beneficial to the organism, and some deleterious (7).
Therefore, a gene may confer a selective advantage within one particular environment, but
its expression could be detrimental within a different environment. Conservation of this gene
ultimately is determined by the overall necessity of the gene to the organism’s fitness.
Bacterial pathogens may evolve mechanisms to neutralize the deleterious effects arising
from antagonistic pleiotropy, while at the same time conserving the beneficial ones.
Temporal regulation is a powerful tool to ensure that specific genes are only turned on when
required, and turned off to prevent detrimental expression within a particular environment.
Certain outer membrane proteins or systems are temporally regulated within the host, as
they may provide a marker for recognition by the host immune system. Flagella expression,
for example, is down-regulated by Salmonella enterica serovar Typhi in vivo to avoid
activation of the host inflammatory response; however, outside the host, motility is likely
important for the bacterium to seek out and scavenge nutrients from the environment (8).
Other bacteria avoid the deleterious effects of a gene through gene inactivation; mutants that
lose functionality of the gene once it becomes deleterious can out-compete the wild type
parent strain, and eventually these mutants will dominate the population. Pseudomonas
Bliven and Maurelli Page 2
Microbiol Spectr. Author manuscript; available in PMC 2016 March 23.
Author Manuscript Author Manuscript Author Manuscript Author Manuscript
population (3). Simply put, allele fixation is prevented because different bacterial alleles
confer distinct advantages to the pathogen in the presence of different host alleles. Evidence
exists within nature for both directional and frequency-dependent selection, and both types
probably occur in bacterial populations.
In this chapter, we explore the host-pathogen interface and offer examples of pathogen
adaptation in response to common host selective pressures (Table 1). Although we will
focus our attention exclusively on bacterial pathogens within the human host, many of the
concepts discussed in this review are readily applicable to other organisms, such as viruses,
parasites, and fungi, which can infect a wide range of hosts including plants, animals, and
amoeba (4-6).
As a final note, much of the evidence presented here to support presumed evolutionary
events is either speculation from what is currently known or suspected about host and
microbial biology, or the result of artificial laboratory-induced evolution during serial
passaging of bacterial strains. Due to the sheer enormity of evolutionary timescales, defining
the precise origins of and factors driving natural evolutionary events is often a difficult
undertaking.
ANTAGONISTIC PLEIOTROPY AND THE FITNESS COST/BENEFIT
ANALYSIS
At the most basic level, the theory of natural selection stipulates that, within a bacterial
population, beneficial traits will be conserved (selected for), and deleterious traits eventually
discarded (selected against). The actual evolutionary process is considerably more complex,
however, due to the existence of genetic drift (the change in genetic diversity of a population
due to random chance) and antagonistic pleiotropy.
Antagonistic pleiotropy is the concept that a single gene may control more than one
phenotype, some of which may be beneficial to the organism, and some deleterious (7).
Therefore, a gene may confer a selective advantage within one particular environment, but
its expression could be detrimental within a different environment. Conservation of this gene
ultimately is determined by the overall necessity of the gene to the organism’s fitness.
Bacterial pathogens may evolve mechanisms to neutralize the deleterious effects arising
from antagonistic pleiotropy, while at the same time conserving the beneficial ones.
Temporal regulation is a powerful tool to ensure that specific genes are only turned on when
required, and turned off to prevent detrimental expression within a particular environment.
Certain outer membrane proteins or systems are temporally regulated within the host, as
they may provide a marker for recognition by the host immune system. Flagella expression,
for example, is down-regulated by Salmonella enterica serovar Typhi in vivo to avoid
activation of the host inflammatory response; however, outside the host, motility is likely
important for the bacterium to seek out and scavenge nutrients from the environment (8).
Other bacteria avoid the deleterious effects of a gene through gene inactivation; mutants that
lose functionality of the gene once it becomes deleterious can out-compete the wild type
parent strain, and eventually these mutants will dominate the population. Pseudomonas
Bliven and Maurelli Page 2
Microbiol Spectr. Author manuscript; available in PMC 2016 March 23.
Author Manuscript Author Manuscript Author Manuscript Author Manuscript
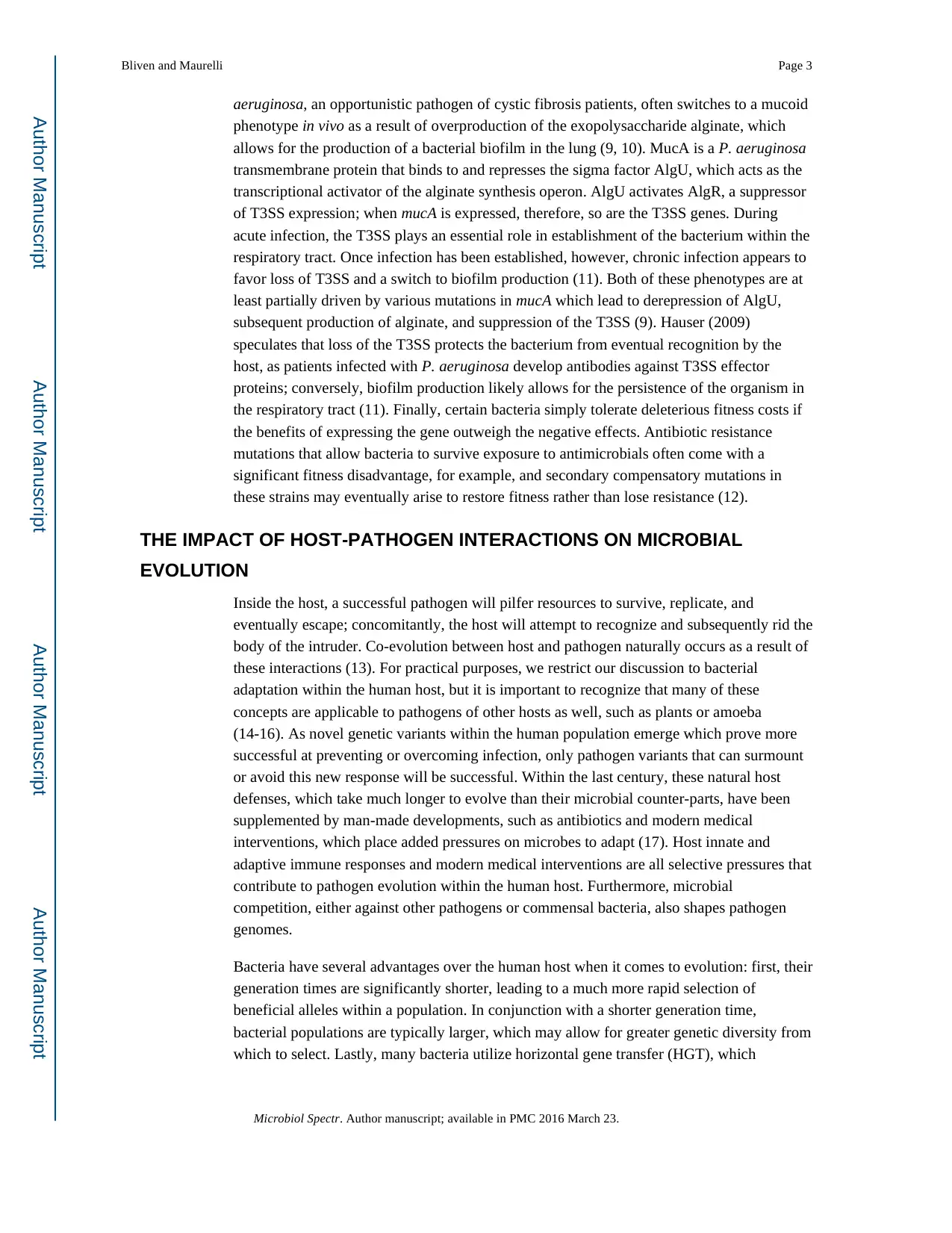
aeruginosa, an opportunistic pathogen of cystic fibrosis patients, often switches to a mucoid
phenotype in vivo as a result of overproduction of the exopolysaccharide alginate, which
allows for the production of a bacterial biofilm in the lung (9, 10). MucA is a P. aeruginosa
transmembrane protein that binds to and represses the sigma factor AlgU, which acts as the
transcriptional activator of the alginate synthesis operon. AlgU activates AlgR, a suppressor
of T3SS expression; when mucA is expressed, therefore, so are the T3SS genes. During
acute infection, the T3SS plays an essential role in establishment of the bacterium within the
respiratory tract. Once infection has been established, however, chronic infection appears to
favor loss of T3SS and a switch to biofilm production (11). Both of these phenotypes are at
least partially driven by various mutations in mucA which lead to derepression of AlgU,
subsequent production of alginate, and suppression of the T3SS (9). Hauser (2009)
speculates that loss of the T3SS protects the bacterium from eventual recognition by the
host, as patients infected with P. aeruginosa develop antibodies against T3SS effector
proteins; conversely, biofilm production likely allows for the persistence of the organism in
the respiratory tract (11). Finally, certain bacteria simply tolerate deleterious fitness costs if
the benefits of expressing the gene outweigh the negative effects. Antibiotic resistance
mutations that allow bacteria to survive exposure to antimicrobials often come with a
significant fitness disadvantage, for example, and secondary compensatory mutations in
these strains may eventually arise to restore fitness rather than lose resistance (12).
THE IMPACT OF HOST-PATHOGEN INTERACTIONS ON MICROBIAL
EVOLUTION
Inside the host, a successful pathogen will pilfer resources to survive, replicate, and
eventually escape; concomitantly, the host will attempt to recognize and subsequently rid the
body of the intruder. Co-evolution between host and pathogen naturally occurs as a result of
these interactions (13). For practical purposes, we restrict our discussion to bacterial
adaptation within the human host, but it is important to recognize that many of these
concepts are applicable to pathogens of other hosts as well, such as plants or amoeba
(14-16). As novel genetic variants within the human population emerge which prove more
successful at preventing or overcoming infection, only pathogen variants that can surmount
or avoid this new response will be successful. Within the last century, these natural host
defenses, which take much longer to evolve than their microbial counter-parts, have been
supplemented by man-made developments, such as antibiotics and modern medical
interventions, which place added pressures on microbes to adapt (17). Host innate and
adaptive immune responses and modern medical interventions are all selective pressures that
contribute to pathogen evolution within the human host. Furthermore, microbial
competition, either against other pathogens or commensal bacteria, also shapes pathogen
genomes.
Bacteria have several advantages over the human host when it comes to evolution: first, their
generation times are significantly shorter, leading to a much more rapid selection of
beneficial alleles within a population. In conjunction with a shorter generation time,
bacterial populations are typically larger, which may allow for greater genetic diversity from
which to select. Lastly, many bacteria utilize horizontal gene transfer (HGT), which
Bliven and Maurelli Page 3
Microbiol Spectr. Author manuscript; available in PMC 2016 March 23.
Author Manuscript Author Manuscript Author Manuscript Author Manuscript
phenotype in vivo as a result of overproduction of the exopolysaccharide alginate, which
allows for the production of a bacterial biofilm in the lung (9, 10). MucA is a P. aeruginosa
transmembrane protein that binds to and represses the sigma factor AlgU, which acts as the
transcriptional activator of the alginate synthesis operon. AlgU activates AlgR, a suppressor
of T3SS expression; when mucA is expressed, therefore, so are the T3SS genes. During
acute infection, the T3SS plays an essential role in establishment of the bacterium within the
respiratory tract. Once infection has been established, however, chronic infection appears to
favor loss of T3SS and a switch to biofilm production (11). Both of these phenotypes are at
least partially driven by various mutations in mucA which lead to derepression of AlgU,
subsequent production of alginate, and suppression of the T3SS (9). Hauser (2009)
speculates that loss of the T3SS protects the bacterium from eventual recognition by the
host, as patients infected with P. aeruginosa develop antibodies against T3SS effector
proteins; conversely, biofilm production likely allows for the persistence of the organism in
the respiratory tract (11). Finally, certain bacteria simply tolerate deleterious fitness costs if
the benefits of expressing the gene outweigh the negative effects. Antibiotic resistance
mutations that allow bacteria to survive exposure to antimicrobials often come with a
significant fitness disadvantage, for example, and secondary compensatory mutations in
these strains may eventually arise to restore fitness rather than lose resistance (12).
THE IMPACT OF HOST-PATHOGEN INTERACTIONS ON MICROBIAL
EVOLUTION
Inside the host, a successful pathogen will pilfer resources to survive, replicate, and
eventually escape; concomitantly, the host will attempt to recognize and subsequently rid the
body of the intruder. Co-evolution between host and pathogen naturally occurs as a result of
these interactions (13). For practical purposes, we restrict our discussion to bacterial
adaptation within the human host, but it is important to recognize that many of these
concepts are applicable to pathogens of other hosts as well, such as plants or amoeba
(14-16). As novel genetic variants within the human population emerge which prove more
successful at preventing or overcoming infection, only pathogen variants that can surmount
or avoid this new response will be successful. Within the last century, these natural host
defenses, which take much longer to evolve than their microbial counter-parts, have been
supplemented by man-made developments, such as antibiotics and modern medical
interventions, which place added pressures on microbes to adapt (17). Host innate and
adaptive immune responses and modern medical interventions are all selective pressures that
contribute to pathogen evolution within the human host. Furthermore, microbial
competition, either against other pathogens or commensal bacteria, also shapes pathogen
genomes.
Bacteria have several advantages over the human host when it comes to evolution: first, their
generation times are significantly shorter, leading to a much more rapid selection of
beneficial alleles within a population. In conjunction with a shorter generation time,
bacterial populations are typically larger, which may allow for greater genetic diversity from
which to select. Lastly, many bacteria utilize horizontal gene transfer (HGT), which
Bliven and Maurelli Page 3
Microbiol Spectr. Author manuscript; available in PMC 2016 March 23.
Author Manuscript Author Manuscript Author Manuscript Author Manuscript
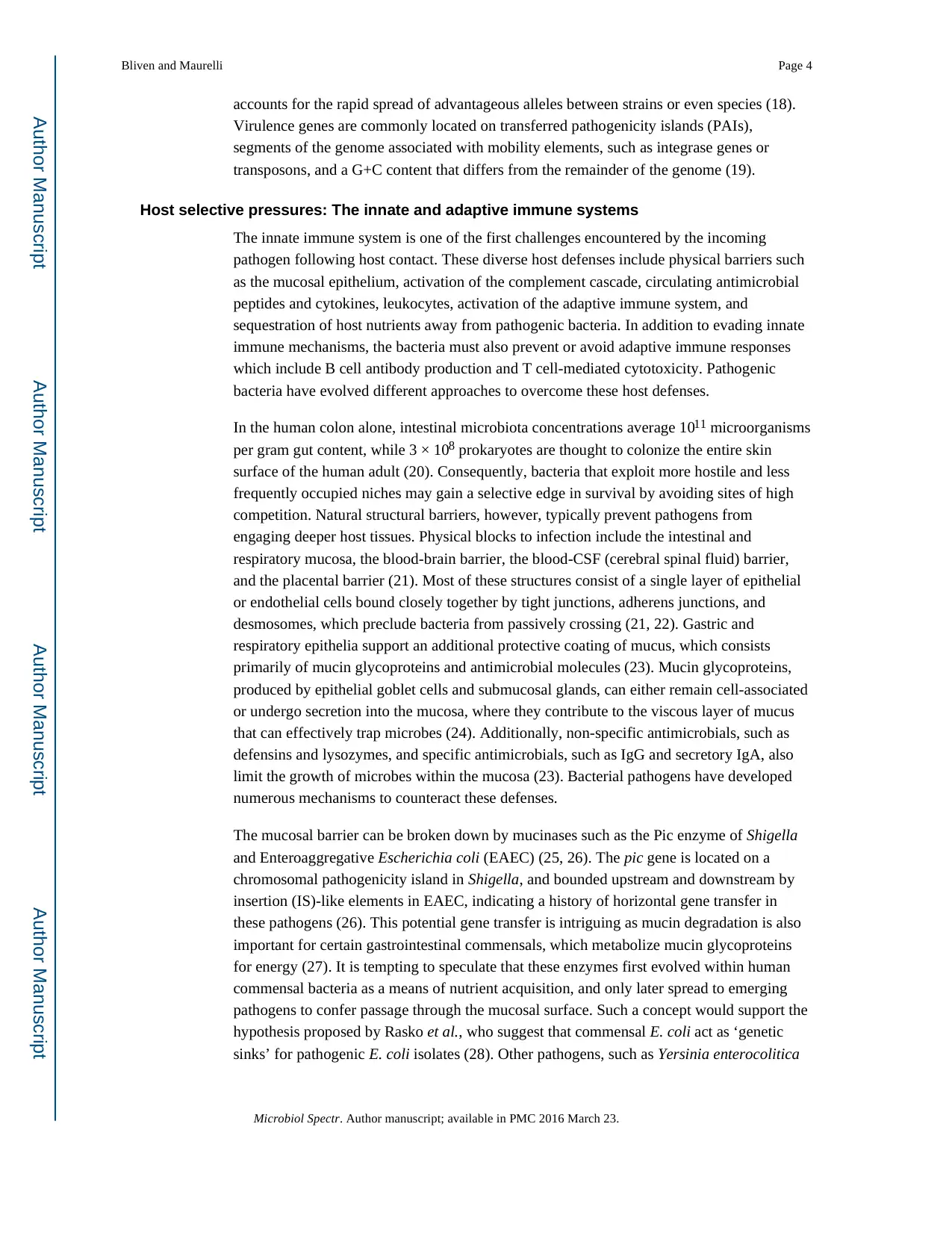
accounts for the rapid spread of advantageous alleles between strains or even species (18).
Virulence genes are commonly located on transferred pathogenicity islands (PAIs),
segments of the genome associated with mobility elements, such as integrase genes or
transposons, and a G+C content that differs from the remainder of the genome (19).
Host selective pressures: The innate and adaptive immune systems
The innate immune system is one of the first challenges encountered by the incoming
pathogen following host contact. These diverse host defenses include physical barriers such
as the mucosal epithelium, activation of the complement cascade, circulating antimicrobial
peptides and cytokines, leukocytes, activation of the adaptive immune system, and
sequestration of host nutrients away from pathogenic bacteria. In addition to evading innate
immune mechanisms, the bacteria must also prevent or avoid adaptive immune responses
which include B cell antibody production and T cell-mediated cytotoxicity. Pathogenic
bacteria have evolved different approaches to overcome these host defenses.
In the human colon alone, intestinal microbiota concentrations average 1011 microorganisms
per gram gut content, while 3 × 108 prokaryotes are thought to colonize the entire skin
surface of the human adult (20). Consequently, bacteria that exploit more hostile and less
frequently occupied niches may gain a selective edge in survival by avoiding sites of high
competition. Natural structural barriers, however, typically prevent pathogens from
engaging deeper host tissues. Physical blocks to infection include the intestinal and
respiratory mucosa, the blood-brain barrier, the blood-CSF (cerebral spinal fluid) barrier,
and the placental barrier (21). Most of these structures consist of a single layer of epithelial
or endothelial cells bound closely together by tight junctions, adherens junctions, and
desmosomes, which preclude bacteria from passively crossing (21, 22). Gastric and
respiratory epithelia support an additional protective coating of mucus, which consists
primarily of mucin glycoproteins and antimicrobial molecules (23). Mucin glycoproteins,
produced by epithelial goblet cells and submucosal glands, can either remain cell-associated
or undergo secretion into the mucosa, where they contribute to the viscous layer of mucus
that can effectively trap microbes (24). Additionally, non-specific antimicrobials, such as
defensins and lysozymes, and specific antimicrobials, such as IgG and secretory IgA, also
limit the growth of microbes within the mucosa (23). Bacterial pathogens have developed
numerous mechanisms to counteract these defenses.
The mucosal barrier can be broken down by mucinases such as the Pic enzyme of Shigella
and Enteroaggregative Escherichia coli (EAEC) (25, 26). The pic gene is located on a
chromosomal pathogenicity island in Shigella, and bounded upstream and downstream by
insertion (IS)-like elements in EAEC, indicating a history of horizontal gene transfer in
these pathogens (26). This potential gene transfer is intriguing as mucin degradation is also
important for certain gastrointestinal commensals, which metabolize mucin glycoproteins
for energy (27). It is tempting to speculate that these enzymes first evolved within human
commensal bacteria as a means of nutrient acquisition, and only later spread to emerging
pathogens to confer passage through the mucosal surface. Such a concept would support the
hypothesis proposed by Rasko et al., who suggest that commensal E. coli act as ‘genetic
sinks’ for pathogenic E. coli isolates (28). Other pathogens, such as Yersinia enterocolitica
Bliven and Maurelli Page 4
Microbiol Spectr. Author manuscript; available in PMC 2016 March 23.
Author Manuscript Author Manuscript Author Manuscript Author Manuscript
Virulence genes are commonly located on transferred pathogenicity islands (PAIs),
segments of the genome associated with mobility elements, such as integrase genes or
transposons, and a G+C content that differs from the remainder of the genome (19).
Host selective pressures: The innate and adaptive immune systems
The innate immune system is one of the first challenges encountered by the incoming
pathogen following host contact. These diverse host defenses include physical barriers such
as the mucosal epithelium, activation of the complement cascade, circulating antimicrobial
peptides and cytokines, leukocytes, activation of the adaptive immune system, and
sequestration of host nutrients away from pathogenic bacteria. In addition to evading innate
immune mechanisms, the bacteria must also prevent or avoid adaptive immune responses
which include B cell antibody production and T cell-mediated cytotoxicity. Pathogenic
bacteria have evolved different approaches to overcome these host defenses.
In the human colon alone, intestinal microbiota concentrations average 1011 microorganisms
per gram gut content, while 3 × 108 prokaryotes are thought to colonize the entire skin
surface of the human adult (20). Consequently, bacteria that exploit more hostile and less
frequently occupied niches may gain a selective edge in survival by avoiding sites of high
competition. Natural structural barriers, however, typically prevent pathogens from
engaging deeper host tissues. Physical blocks to infection include the intestinal and
respiratory mucosa, the blood-brain barrier, the blood-CSF (cerebral spinal fluid) barrier,
and the placental barrier (21). Most of these structures consist of a single layer of epithelial
or endothelial cells bound closely together by tight junctions, adherens junctions, and
desmosomes, which preclude bacteria from passively crossing (21, 22). Gastric and
respiratory epithelia support an additional protective coating of mucus, which consists
primarily of mucin glycoproteins and antimicrobial molecules (23). Mucin glycoproteins,
produced by epithelial goblet cells and submucosal glands, can either remain cell-associated
or undergo secretion into the mucosa, where they contribute to the viscous layer of mucus
that can effectively trap microbes (24). Additionally, non-specific antimicrobials, such as
defensins and lysozymes, and specific antimicrobials, such as IgG and secretory IgA, also
limit the growth of microbes within the mucosa (23). Bacterial pathogens have developed
numerous mechanisms to counteract these defenses.
The mucosal barrier can be broken down by mucinases such as the Pic enzyme of Shigella
and Enteroaggregative Escherichia coli (EAEC) (25, 26). The pic gene is located on a
chromosomal pathogenicity island in Shigella, and bounded upstream and downstream by
insertion (IS)-like elements in EAEC, indicating a history of horizontal gene transfer in
these pathogens (26). This potential gene transfer is intriguing as mucin degradation is also
important for certain gastrointestinal commensals, which metabolize mucin glycoproteins
for energy (27). It is tempting to speculate that these enzymes first evolved within human
commensal bacteria as a means of nutrient acquisition, and only later spread to emerging
pathogens to confer passage through the mucosal surface. Such a concept would support the
hypothesis proposed by Rasko et al., who suggest that commensal E. coli act as ‘genetic
sinks’ for pathogenic E. coli isolates (28). Other pathogens, such as Yersinia enterocolitica
Bliven and Maurelli Page 4
Microbiol Spectr. Author manuscript; available in PMC 2016 March 23.
Author Manuscript Author Manuscript Author Manuscript Author Manuscript
Secure Best Marks with AI Grader
Need help grading? Try our AI Grader for instant feedback on your assignments.
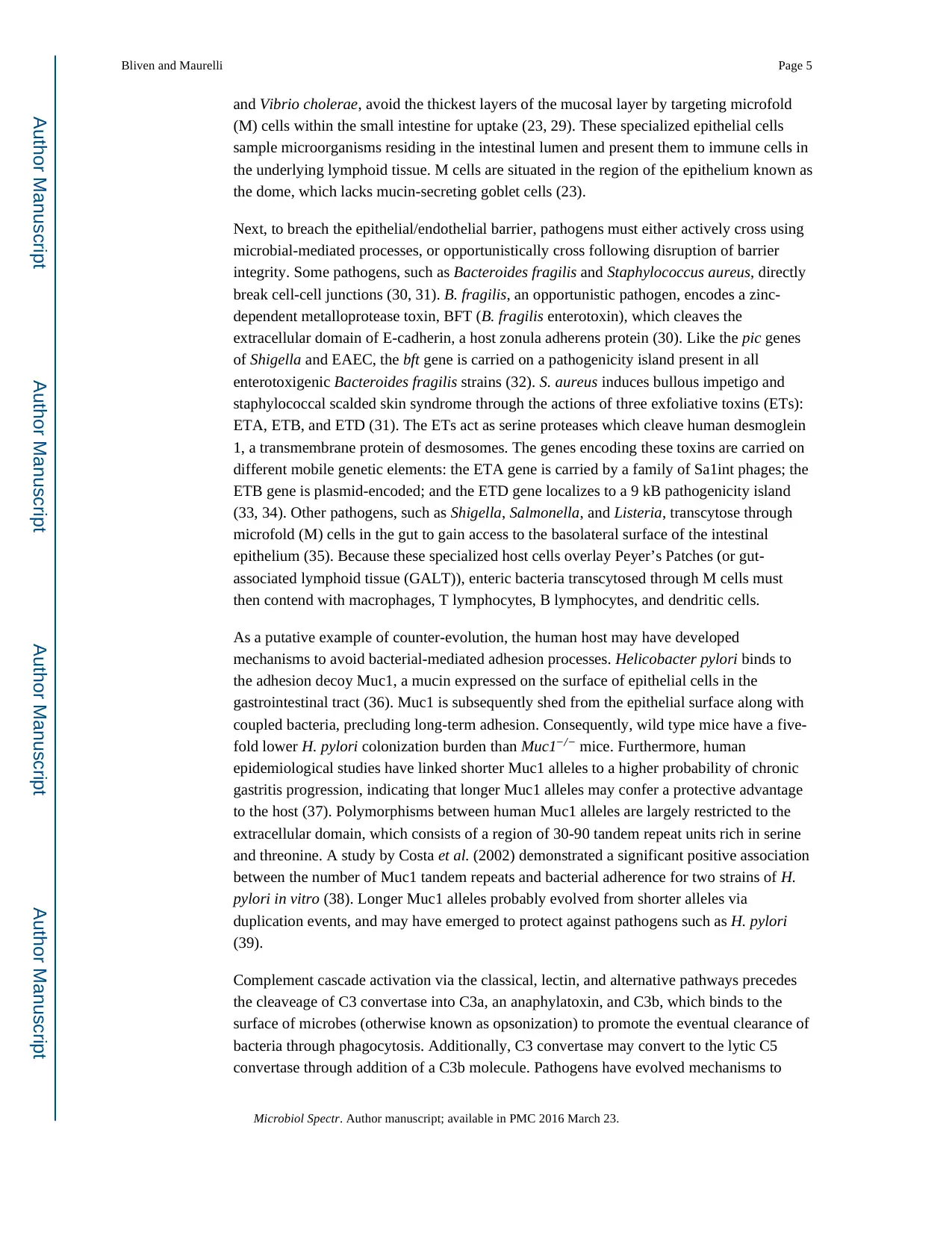
and Vibrio cholerae, avoid the thickest layers of the mucosal layer by targeting microfold
(M) cells within the small intestine for uptake (23, 29). These specialized epithelial cells
sample microorganisms residing in the intestinal lumen and present them to immune cells in
the underlying lymphoid tissue. M cells are situated in the region of the epithelium known as
the dome, which lacks mucin-secreting goblet cells (23).
Next, to breach the epithelial/endothelial barrier, pathogens must either actively cross using
microbial-mediated processes, or opportunistically cross following disruption of barrier
integrity. Some pathogens, such as Bacteroides fragilis and Staphylococcus aureus, directly
break cell-cell junctions (30, 31). B. fragilis, an opportunistic pathogen, encodes a zinc-
dependent metalloprotease toxin, BFT (B. fragilis enterotoxin), which cleaves the
extracellular domain of E-cadherin, a host zonula adherens protein (30). Like the pic genes
of Shigella and EAEC, the bft gene is carried on a pathogenicity island present in all
enterotoxigenic Bacteroides fragilis strains (32). S. aureus induces bullous impetigo and
staphylococcal scalded skin syndrome through the actions of three exfoliative toxins (ETs):
ETA, ETB, and ETD (31). The ETs act as serine proteases which cleave human desmoglein
1, a transmembrane protein of desmosomes. The genes encoding these toxins are carried on
different mobile genetic elements: the ETA gene is carried by a family of Sa1int phages; the
ETB gene is plasmid-encoded; and the ETD gene localizes to a 9 kB pathogenicity island
(33, 34). Other pathogens, such as Shigella, Salmonella, and Listeria, transcytose through
microfold (M) cells in the gut to gain access to the basolateral surface of the intestinal
epithelium (35). Because these specialized host cells overlay Peyer’s Patches (or gut-
associated lymphoid tissue (GALT)), enteric bacteria transcytosed through M cells must
then contend with macrophages, T lymphocytes, B lymphocytes, and dendritic cells.
As a putative example of counter-evolution, the human host may have developed
mechanisms to avoid bacterial-mediated adhesion processes. Helicobacter pylori binds to
the adhesion decoy Muc1, a mucin expressed on the surface of epithelial cells in the
gastrointestinal tract (36). Muc1 is subsequently shed from the epithelial surface along with
coupled bacteria, precluding long-term adhesion. Consequently, wild type mice have a five-
fold lower H. pylori colonization burden than Muc1−/− mice. Furthermore, human
epidemiological studies have linked shorter Muc1 alleles to a higher probability of chronic
gastritis progression, indicating that longer Muc1 alleles may confer a protective advantage
to the host (37). Polymorphisms between human Muc1 alleles are largely restricted to the
extracellular domain, which consists of a region of 30-90 tandem repeat units rich in serine
and threonine. A study by Costa et al. (2002) demonstrated a significant positive association
between the number of Muc1 tandem repeats and bacterial adherence for two strains of H.
pylori in vitro (38). Longer Muc1 alleles probably evolved from shorter alleles via
duplication events, and may have emerged to protect against pathogens such as H. pylori
(39).
Complement cascade activation via the classical, lectin, and alternative pathways precedes
the cleaveage of C3 convertase into C3a, an anaphylatoxin, and C3b, which binds to the
surface of microbes (otherwise known as opsonization) to promote the eventual clearance of
bacteria through phagocytosis. Additionally, C3 convertase may convert to the lytic C5
convertase through addition of a C3b molecule. Pathogens have evolved mechanisms to
Bliven and Maurelli Page 5
Microbiol Spectr. Author manuscript; available in PMC 2016 March 23.
Author Manuscript Author Manuscript Author Manuscript Author Manuscript
(M) cells within the small intestine for uptake (23, 29). These specialized epithelial cells
sample microorganisms residing in the intestinal lumen and present them to immune cells in
the underlying lymphoid tissue. M cells are situated in the region of the epithelium known as
the dome, which lacks mucin-secreting goblet cells (23).
Next, to breach the epithelial/endothelial barrier, pathogens must either actively cross using
microbial-mediated processes, or opportunistically cross following disruption of barrier
integrity. Some pathogens, such as Bacteroides fragilis and Staphylococcus aureus, directly
break cell-cell junctions (30, 31). B. fragilis, an opportunistic pathogen, encodes a zinc-
dependent metalloprotease toxin, BFT (B. fragilis enterotoxin), which cleaves the
extracellular domain of E-cadherin, a host zonula adherens protein (30). Like the pic genes
of Shigella and EAEC, the bft gene is carried on a pathogenicity island present in all
enterotoxigenic Bacteroides fragilis strains (32). S. aureus induces bullous impetigo and
staphylococcal scalded skin syndrome through the actions of three exfoliative toxins (ETs):
ETA, ETB, and ETD (31). The ETs act as serine proteases which cleave human desmoglein
1, a transmembrane protein of desmosomes. The genes encoding these toxins are carried on
different mobile genetic elements: the ETA gene is carried by a family of Sa1int phages; the
ETB gene is plasmid-encoded; and the ETD gene localizes to a 9 kB pathogenicity island
(33, 34). Other pathogens, such as Shigella, Salmonella, and Listeria, transcytose through
microfold (M) cells in the gut to gain access to the basolateral surface of the intestinal
epithelium (35). Because these specialized host cells overlay Peyer’s Patches (or gut-
associated lymphoid tissue (GALT)), enteric bacteria transcytosed through M cells must
then contend with macrophages, T lymphocytes, B lymphocytes, and dendritic cells.
As a putative example of counter-evolution, the human host may have developed
mechanisms to avoid bacterial-mediated adhesion processes. Helicobacter pylori binds to
the adhesion decoy Muc1, a mucin expressed on the surface of epithelial cells in the
gastrointestinal tract (36). Muc1 is subsequently shed from the epithelial surface along with
coupled bacteria, precluding long-term adhesion. Consequently, wild type mice have a five-
fold lower H. pylori colonization burden than Muc1−/− mice. Furthermore, human
epidemiological studies have linked shorter Muc1 alleles to a higher probability of chronic
gastritis progression, indicating that longer Muc1 alleles may confer a protective advantage
to the host (37). Polymorphisms between human Muc1 alleles are largely restricted to the
extracellular domain, which consists of a region of 30-90 tandem repeat units rich in serine
and threonine. A study by Costa et al. (2002) demonstrated a significant positive association
between the number of Muc1 tandem repeats and bacterial adherence for two strains of H.
pylori in vitro (38). Longer Muc1 alleles probably evolved from shorter alleles via
duplication events, and may have emerged to protect against pathogens such as H. pylori
(39).
Complement cascade activation via the classical, lectin, and alternative pathways precedes
the cleaveage of C3 convertase into C3a, an anaphylatoxin, and C3b, which binds to the
surface of microbes (otherwise known as opsonization) to promote the eventual clearance of
bacteria through phagocytosis. Additionally, C3 convertase may convert to the lytic C5
convertase through addition of a C3b molecule. Pathogens have evolved mechanisms to
Bliven and Maurelli Page 5
Microbiol Spectr. Author manuscript; available in PMC 2016 March 23.
Author Manuscript Author Manuscript Author Manuscript Author Manuscript
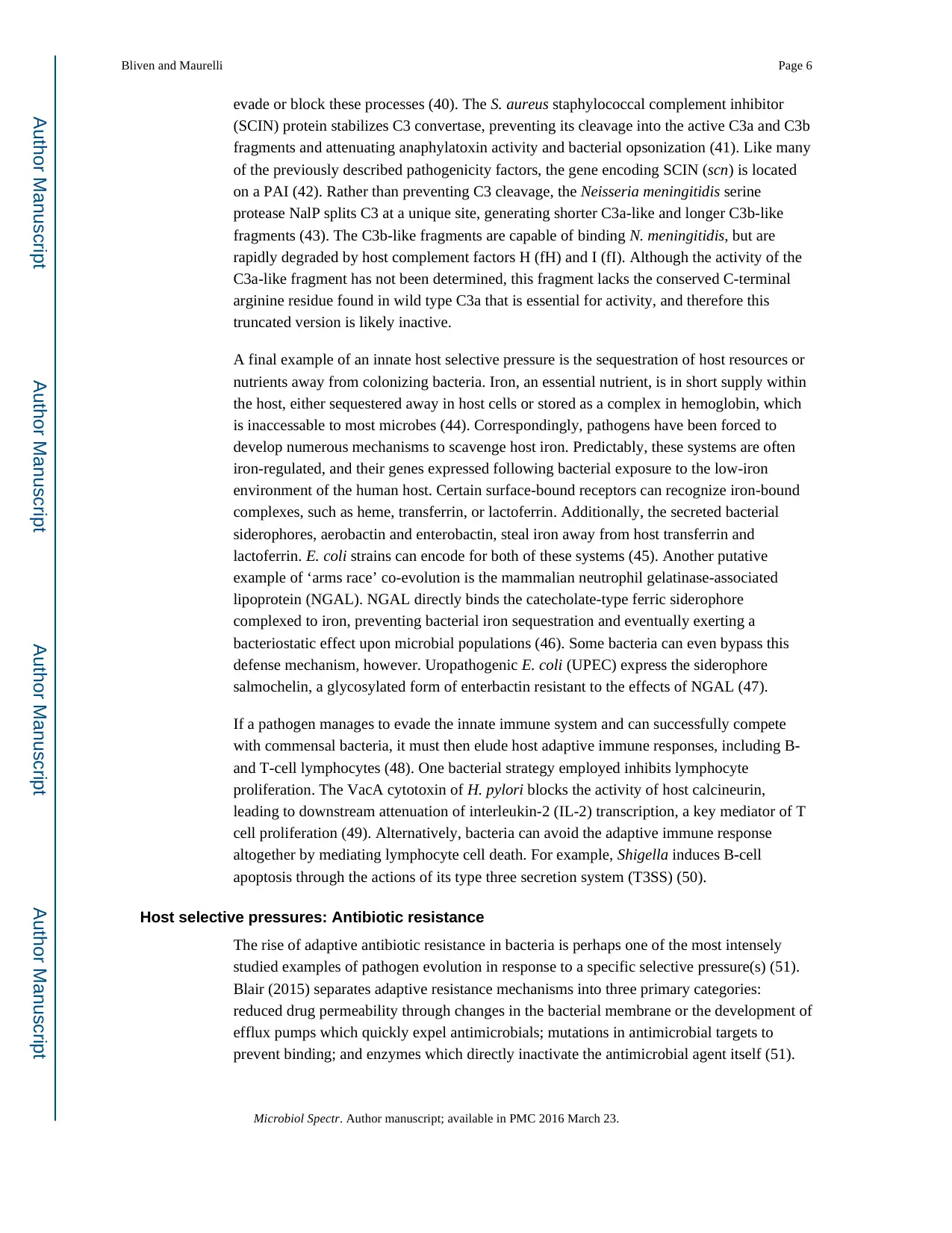
evade or block these processes (40). The S. aureus staphylococcal complement inhibitor
(SCIN) protein stabilizes C3 convertase, preventing its cleavage into the active C3a and C3b
fragments and attenuating anaphylatoxin activity and bacterial opsonization (41). Like many
of the previously described pathogenicity factors, the gene encoding SCIN (scn) is located
on a PAI (42). Rather than preventing C3 cleavage, the Neisseria meningitidis serine
protease NalP splits C3 at a unique site, generating shorter C3a-like and longer C3b-like
fragments (43). The C3b-like fragments are capable of binding N. meningitidis, but are
rapidly degraded by host complement factors H (fH) and I (fI). Although the activity of the
C3a-like fragment has not been determined, this fragment lacks the conserved C-terminal
arginine residue found in wild type C3a that is essential for activity, and therefore this
truncated version is likely inactive.
A final example of an innate host selective pressure is the sequestration of host resources or
nutrients away from colonizing bacteria. Iron, an essential nutrient, is in short supply within
the host, either sequestered away in host cells or stored as a complex in hemoglobin, which
is inaccessable to most microbes (44). Correspondingly, pathogens have been forced to
develop numerous mechanisms to scavenge host iron. Predictably, these systems are often
iron-regulated, and their genes expressed following bacterial exposure to the low-iron
environment of the human host. Certain surface-bound receptors can recognize iron-bound
complexes, such as heme, transferrin, or lactoferrin. Additionally, the secreted bacterial
siderophores, aerobactin and enterobactin, steal iron away from host transferrin and
lactoferrin. E. coli strains can encode for both of these systems (45). Another putative
example of ‘arms race’ co-evolution is the mammalian neutrophil gelatinase-associated
lipoprotein (NGAL). NGAL directly binds the catecholate-type ferric siderophore
complexed to iron, preventing bacterial iron sequestration and eventually exerting a
bacteriostatic effect upon microbial populations (46). Some bacteria can even bypass this
defense mechanism, however. Uropathogenic E. coli (UPEC) express the siderophore
salmochelin, a glycosylated form of enterbactin resistant to the effects of NGAL (47).
If a pathogen manages to evade the innate immune system and can successfully compete
with commensal bacteria, it must then elude host adaptive immune responses, including B-
and T-cell lymphocytes (48). One bacterial strategy employed inhibits lymphocyte
proliferation. The VacA cytotoxin of H. pylori blocks the activity of host calcineurin,
leading to downstream attenuation of interleukin-2 (IL-2) transcription, a key mediator of T
cell proliferation (49). Alternatively, bacteria can avoid the adaptive immune response
altogether by mediating lymphocyte cell death. For example, Shigella induces B-cell
apoptosis through the actions of its type three secretion system (T3SS) (50).
Host selective pressures: Antibiotic resistance
The rise of adaptive antibiotic resistance in bacteria is perhaps one of the most intensely
studied examples of pathogen evolution in response to a specific selective pressure(s) (51).
Blair (2015) separates adaptive resistance mechanisms into three primary categories:
reduced drug permeability through changes in the bacterial membrane or the development of
efflux pumps which quickly expel antimicrobials; mutations in antimicrobial targets to
prevent binding; and enzymes which directly inactivate the antimicrobial agent itself (51).
Bliven and Maurelli Page 6
Microbiol Spectr. Author manuscript; available in PMC 2016 March 23.
Author Manuscript Author Manuscript Author Manuscript Author Manuscript
(SCIN) protein stabilizes C3 convertase, preventing its cleavage into the active C3a and C3b
fragments and attenuating anaphylatoxin activity and bacterial opsonization (41). Like many
of the previously described pathogenicity factors, the gene encoding SCIN (scn) is located
on a PAI (42). Rather than preventing C3 cleavage, the Neisseria meningitidis serine
protease NalP splits C3 at a unique site, generating shorter C3a-like and longer C3b-like
fragments (43). The C3b-like fragments are capable of binding N. meningitidis, but are
rapidly degraded by host complement factors H (fH) and I (fI). Although the activity of the
C3a-like fragment has not been determined, this fragment lacks the conserved C-terminal
arginine residue found in wild type C3a that is essential for activity, and therefore this
truncated version is likely inactive.
A final example of an innate host selective pressure is the sequestration of host resources or
nutrients away from colonizing bacteria. Iron, an essential nutrient, is in short supply within
the host, either sequestered away in host cells or stored as a complex in hemoglobin, which
is inaccessable to most microbes (44). Correspondingly, pathogens have been forced to
develop numerous mechanisms to scavenge host iron. Predictably, these systems are often
iron-regulated, and their genes expressed following bacterial exposure to the low-iron
environment of the human host. Certain surface-bound receptors can recognize iron-bound
complexes, such as heme, transferrin, or lactoferrin. Additionally, the secreted bacterial
siderophores, aerobactin and enterobactin, steal iron away from host transferrin and
lactoferrin. E. coli strains can encode for both of these systems (45). Another putative
example of ‘arms race’ co-evolution is the mammalian neutrophil gelatinase-associated
lipoprotein (NGAL). NGAL directly binds the catecholate-type ferric siderophore
complexed to iron, preventing bacterial iron sequestration and eventually exerting a
bacteriostatic effect upon microbial populations (46). Some bacteria can even bypass this
defense mechanism, however. Uropathogenic E. coli (UPEC) express the siderophore
salmochelin, a glycosylated form of enterbactin resistant to the effects of NGAL (47).
If a pathogen manages to evade the innate immune system and can successfully compete
with commensal bacteria, it must then elude host adaptive immune responses, including B-
and T-cell lymphocytes (48). One bacterial strategy employed inhibits lymphocyte
proliferation. The VacA cytotoxin of H. pylori blocks the activity of host calcineurin,
leading to downstream attenuation of interleukin-2 (IL-2) transcription, a key mediator of T
cell proliferation (49). Alternatively, bacteria can avoid the adaptive immune response
altogether by mediating lymphocyte cell death. For example, Shigella induces B-cell
apoptosis through the actions of its type three secretion system (T3SS) (50).
Host selective pressures: Antibiotic resistance
The rise of adaptive antibiotic resistance in bacteria is perhaps one of the most intensely
studied examples of pathogen evolution in response to a specific selective pressure(s) (51).
Blair (2015) separates adaptive resistance mechanisms into three primary categories:
reduced drug permeability through changes in the bacterial membrane or the development of
efflux pumps which quickly expel antimicrobials; mutations in antimicrobial targets to
prevent binding; and enzymes which directly inactivate the antimicrobial agent itself (51).
Bliven and Maurelli Page 6
Microbiol Spectr. Author manuscript; available in PMC 2016 March 23.
Author Manuscript Author Manuscript Author Manuscript Author Manuscript
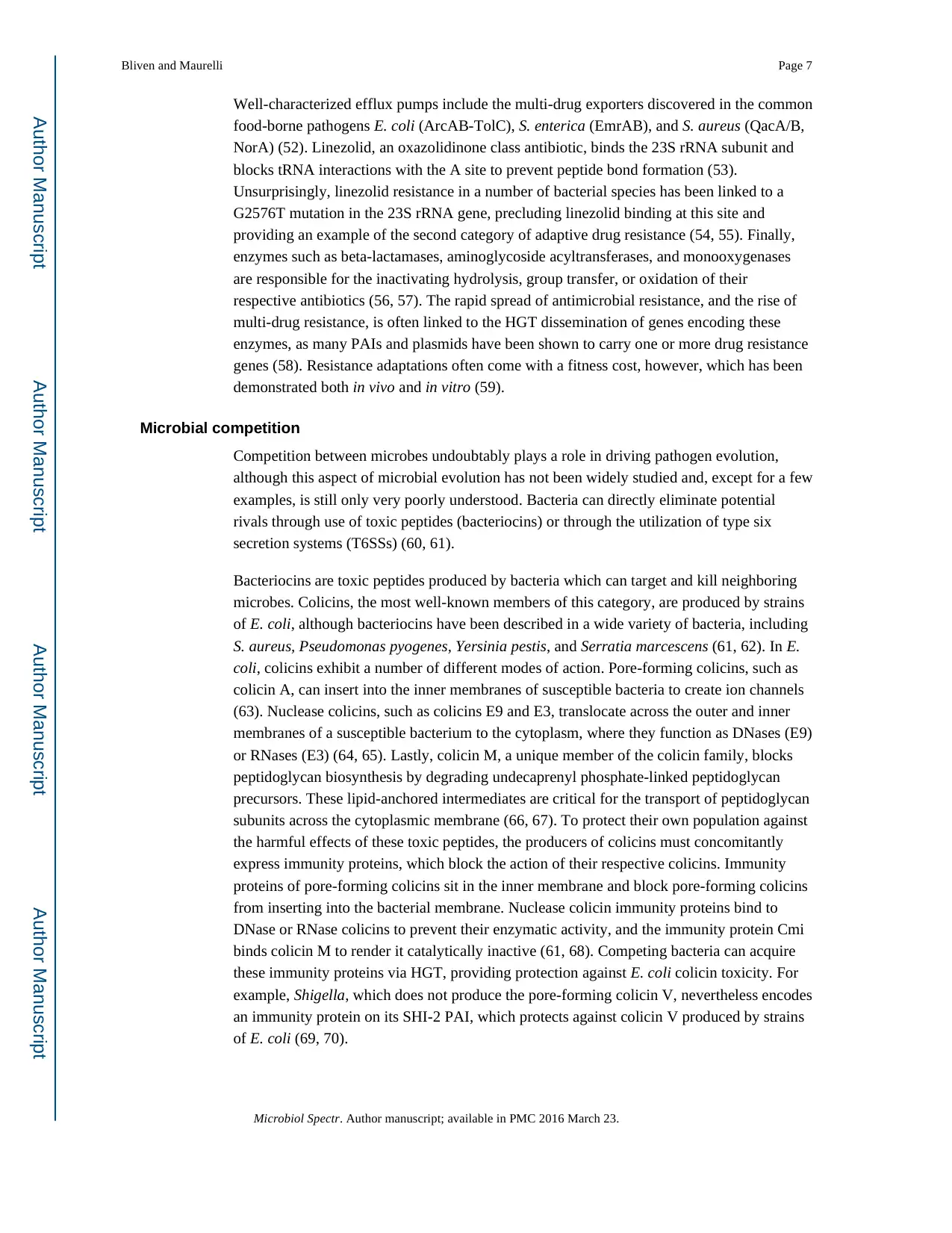
Well-characterized efflux pumps include the multi-drug exporters discovered in the common
food-borne pathogens E. coli (ArcAB-TolC), S. enterica (EmrAB), and S. aureus (QacA/B,
NorA) (52). Linezolid, an oxazolidinone class antibiotic, binds the 23S rRNA subunit and
blocks tRNA interactions with the A site to prevent peptide bond formation (53).
Unsurprisingly, linezolid resistance in a number of bacterial species has been linked to a
G2576T mutation in the 23S rRNA gene, precluding linezolid binding at this site and
providing an example of the second category of adaptive drug resistance (54, 55). Finally,
enzymes such as beta-lactamases, aminoglycoside acyltransferases, and monooxygenases
are responsible for the inactivating hydrolysis, group transfer, or oxidation of their
respective antibiotics (56, 57). The rapid spread of antimicrobial resistance, and the rise of
multi-drug resistance, is often linked to the HGT dissemination of genes encoding these
enzymes, as many PAIs and plasmids have been shown to carry one or more drug resistance
genes (58). Resistance adaptations often come with a fitness cost, however, which has been
demonstrated both in vivo and in vitro (59).
Microbial competition
Competition between microbes undoubtably plays a role in driving pathogen evolution,
although this aspect of microbial evolution has not been widely studied and, except for a few
examples, is still only very poorly understood. Bacteria can directly eliminate potential
rivals through use of toxic peptides (bacteriocins) or through the utilization of type six
secretion systems (T6SSs) (60, 61).
Bacteriocins are toxic peptides produced by bacteria which can target and kill neighboring
microbes. Colicins, the most well-known members of this category, are produced by strains
of E. coli, although bacteriocins have been described in a wide variety of bacteria, including
S. aureus, Pseudomonas pyogenes, Yersinia pestis, and Serratia marcescens (61, 62). In E.
coli, colicins exhibit a number of different modes of action. Pore-forming colicins, such as
colicin A, can insert into the inner membranes of susceptible bacteria to create ion channels
(63). Nuclease colicins, such as colicins E9 and E3, translocate across the outer and inner
membranes of a susceptible bacterium to the cytoplasm, where they function as DNases (E9)
or RNases (E3) (64, 65). Lastly, colicin M, a unique member of the colicin family, blocks
peptidoglycan biosynthesis by degrading undecaprenyl phosphate-linked peptidoglycan
precursors. These lipid-anchored intermediates are critical for the transport of peptidoglycan
subunits across the cytoplasmic membrane (66, 67). To protect their own population against
the harmful effects of these toxic peptides, the producers of colicins must concomitantly
express immunity proteins, which block the action of their respective colicins. Immunity
proteins of pore-forming colicins sit in the inner membrane and block pore-forming colicins
from inserting into the bacterial membrane. Nuclease colicin immunity proteins bind to
DNase or RNase colicins to prevent their enzymatic activity, and the immunity protein Cmi
binds colicin M to render it catalytically inactive (61, 68). Competing bacteria can acquire
these immunity proteins via HGT, providing protection against E. coli colicin toxicity. For
example, Shigella, which does not produce the pore-forming colicin V, nevertheless encodes
an immunity protein on its SHI-2 PAI, which protects against colicin V produced by strains
of E. coli (69, 70).
Bliven and Maurelli Page 7
Microbiol Spectr. Author manuscript; available in PMC 2016 March 23.
Author Manuscript Author Manuscript Author Manuscript Author Manuscript
food-borne pathogens E. coli (ArcAB-TolC), S. enterica (EmrAB), and S. aureus (QacA/B,
NorA) (52). Linezolid, an oxazolidinone class antibiotic, binds the 23S rRNA subunit and
blocks tRNA interactions with the A site to prevent peptide bond formation (53).
Unsurprisingly, linezolid resistance in a number of bacterial species has been linked to a
G2576T mutation in the 23S rRNA gene, precluding linezolid binding at this site and
providing an example of the second category of adaptive drug resistance (54, 55). Finally,
enzymes such as beta-lactamases, aminoglycoside acyltransferases, and monooxygenases
are responsible for the inactivating hydrolysis, group transfer, or oxidation of their
respective antibiotics (56, 57). The rapid spread of antimicrobial resistance, and the rise of
multi-drug resistance, is often linked to the HGT dissemination of genes encoding these
enzymes, as many PAIs and plasmids have been shown to carry one or more drug resistance
genes (58). Resistance adaptations often come with a fitness cost, however, which has been
demonstrated both in vivo and in vitro (59).
Microbial competition
Competition between microbes undoubtably plays a role in driving pathogen evolution,
although this aspect of microbial evolution has not been widely studied and, except for a few
examples, is still only very poorly understood. Bacteria can directly eliminate potential
rivals through use of toxic peptides (bacteriocins) or through the utilization of type six
secretion systems (T6SSs) (60, 61).
Bacteriocins are toxic peptides produced by bacteria which can target and kill neighboring
microbes. Colicins, the most well-known members of this category, are produced by strains
of E. coli, although bacteriocins have been described in a wide variety of bacteria, including
S. aureus, Pseudomonas pyogenes, Yersinia pestis, and Serratia marcescens (61, 62). In E.
coli, colicins exhibit a number of different modes of action. Pore-forming colicins, such as
colicin A, can insert into the inner membranes of susceptible bacteria to create ion channels
(63). Nuclease colicins, such as colicins E9 and E3, translocate across the outer and inner
membranes of a susceptible bacterium to the cytoplasm, where they function as DNases (E9)
or RNases (E3) (64, 65). Lastly, colicin M, a unique member of the colicin family, blocks
peptidoglycan biosynthesis by degrading undecaprenyl phosphate-linked peptidoglycan
precursors. These lipid-anchored intermediates are critical for the transport of peptidoglycan
subunits across the cytoplasmic membrane (66, 67). To protect their own population against
the harmful effects of these toxic peptides, the producers of colicins must concomitantly
express immunity proteins, which block the action of their respective colicins. Immunity
proteins of pore-forming colicins sit in the inner membrane and block pore-forming colicins
from inserting into the bacterial membrane. Nuclease colicin immunity proteins bind to
DNase or RNase colicins to prevent their enzymatic activity, and the immunity protein Cmi
binds colicin M to render it catalytically inactive (61, 68). Competing bacteria can acquire
these immunity proteins via HGT, providing protection against E. coli colicin toxicity. For
example, Shigella, which does not produce the pore-forming colicin V, nevertheless encodes
an immunity protein on its SHI-2 PAI, which protects against colicin V produced by strains
of E. coli (69, 70).
Bliven and Maurelli Page 7
Microbiol Spectr. Author manuscript; available in PMC 2016 March 23.
Author Manuscript Author Manuscript Author Manuscript Author Manuscript
Paraphrase This Document
Need a fresh take? Get an instant paraphrase of this document with our AI Paraphraser
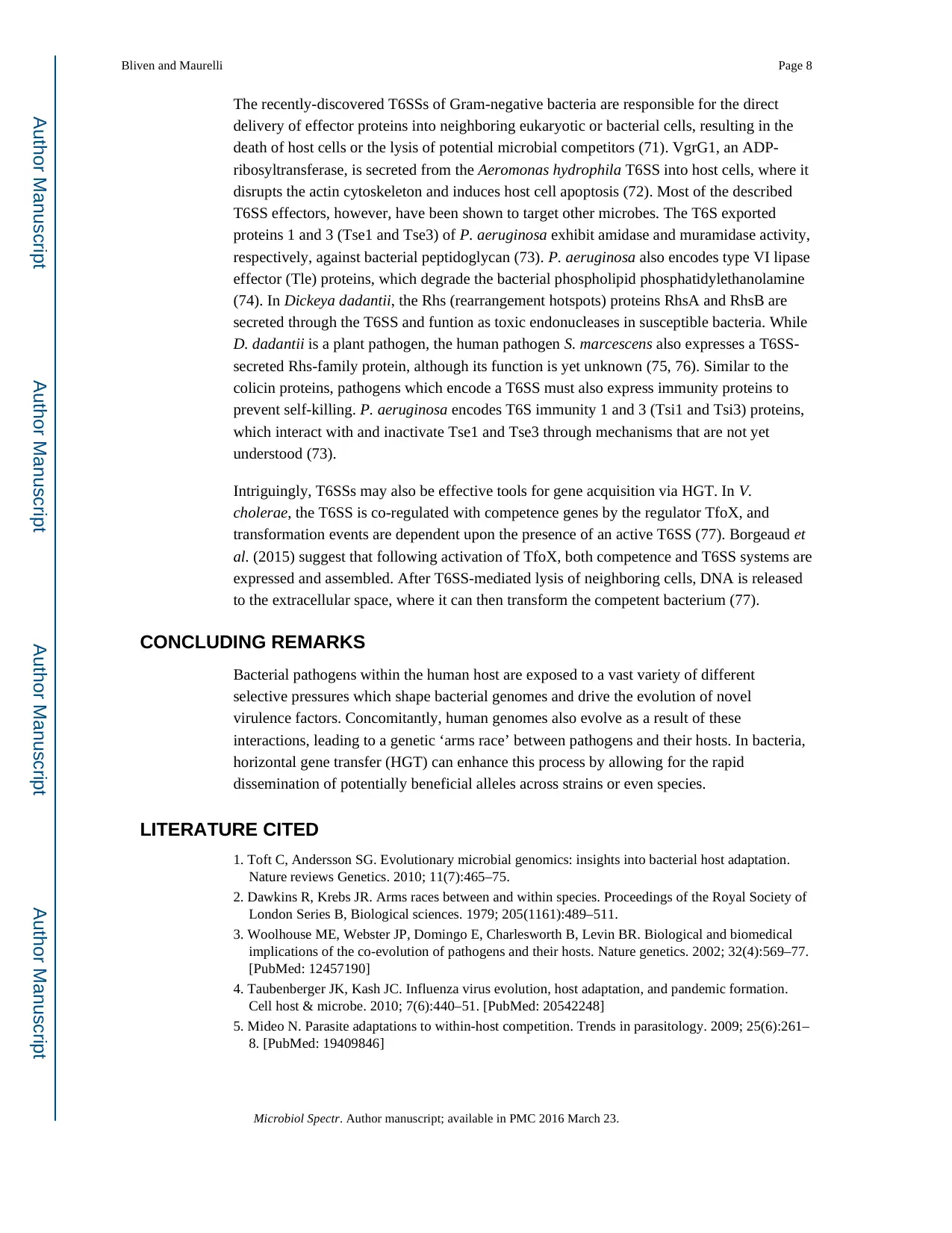
The recently-discovered T6SSs of Gram-negative bacteria are responsible for the direct
delivery of effector proteins into neighboring eukaryotic or bacterial cells, resulting in the
death of host cells or the lysis of potential microbial competitors (71). VgrG1, an ADP-
ribosyltransferase, is secreted from the Aeromonas hydrophila T6SS into host cells, where it
disrupts the actin cytoskeleton and induces host cell apoptosis (72). Most of the described
T6SS effectors, however, have been shown to target other microbes. The T6S exported
proteins 1 and 3 (Tse1 and Tse3) of P. aeruginosa exhibit amidase and muramidase activity,
respectively, against bacterial peptidoglycan (73). P. aeruginosa also encodes type VI lipase
effector (Tle) proteins, which degrade the bacterial phospholipid phosphatidylethanolamine
(74). In Dickeya dadantii, the Rhs (rearrangement hotspots) proteins RhsA and RhsB are
secreted through the T6SS and funtion as toxic endonucleases in susceptible bacteria. While
D. dadantii is a plant pathogen, the human pathogen S. marcescens also expresses a T6SS-
secreted Rhs-family protein, although its function is yet unknown (75, 76). Similar to the
colicin proteins, pathogens which encode a T6SS must also express immunity proteins to
prevent self-killing. P. aeruginosa encodes T6S immunity 1 and 3 (Tsi1 and Tsi3) proteins,
which interact with and inactivate Tse1 and Tse3 through mechanisms that are not yet
understood (73).
Intriguingly, T6SSs may also be effective tools for gene acquisition via HGT. In V.
cholerae, the T6SS is co-regulated with competence genes by the regulator TfoX, and
transformation events are dependent upon the presence of an active T6SS (77). Borgeaud et
al. (2015) suggest that following activation of TfoX, both competence and T6SS systems are
expressed and assembled. After T6SS-mediated lysis of neighboring cells, DNA is released
to the extracellular space, where it can then transform the competent bacterium (77).
CONCLUDING REMARKS
Bacterial pathogens within the human host are exposed to a vast variety of different
selective pressures which shape bacterial genomes and drive the evolution of novel
virulence factors. Concomitantly, human genomes also evolve as a result of these
interactions, leading to a genetic ‘arms race’ between pathogens and their hosts. In bacteria,
horizontal gene transfer (HGT) can enhance this process by allowing for the rapid
dissemination of potentially beneficial alleles across strains or even species.
LITERATURE CITED
1. Toft C, Andersson SG. Evolutionary microbial genomics: insights into bacterial host adaptation.
Nature reviews Genetics. 2010; 11(7):465–75.
2. Dawkins R, Krebs JR. Arms races between and within species. Proceedings of the Royal Society of
London Series B, Biological sciences. 1979; 205(1161):489–511.
3. Woolhouse ME, Webster JP, Domingo E, Charlesworth B, Levin BR. Biological and biomedical
implications of the co-evolution of pathogens and their hosts. Nature genetics. 2002; 32(4):569–77.
[PubMed: 12457190]
4. Taubenberger JK, Kash JC. Influenza virus evolution, host adaptation, and pandemic formation.
Cell host & microbe. 2010; 7(6):440–51. [PubMed: 20542248]
5. Mideo N. Parasite adaptations to within-host competition. Trends in parasitology. 2009; 25(6):261–
8. [PubMed: 19409846]
Bliven and Maurelli Page 8
Microbiol Spectr. Author manuscript; available in PMC 2016 March 23.
Author Manuscript Author Manuscript Author Manuscript Author Manuscript
delivery of effector proteins into neighboring eukaryotic or bacterial cells, resulting in the
death of host cells or the lysis of potential microbial competitors (71). VgrG1, an ADP-
ribosyltransferase, is secreted from the Aeromonas hydrophila T6SS into host cells, where it
disrupts the actin cytoskeleton and induces host cell apoptosis (72). Most of the described
T6SS effectors, however, have been shown to target other microbes. The T6S exported
proteins 1 and 3 (Tse1 and Tse3) of P. aeruginosa exhibit amidase and muramidase activity,
respectively, against bacterial peptidoglycan (73). P. aeruginosa also encodes type VI lipase
effector (Tle) proteins, which degrade the bacterial phospholipid phosphatidylethanolamine
(74). In Dickeya dadantii, the Rhs (rearrangement hotspots) proteins RhsA and RhsB are
secreted through the T6SS and funtion as toxic endonucleases in susceptible bacteria. While
D. dadantii is a plant pathogen, the human pathogen S. marcescens also expresses a T6SS-
secreted Rhs-family protein, although its function is yet unknown (75, 76). Similar to the
colicin proteins, pathogens which encode a T6SS must also express immunity proteins to
prevent self-killing. P. aeruginosa encodes T6S immunity 1 and 3 (Tsi1 and Tsi3) proteins,
which interact with and inactivate Tse1 and Tse3 through mechanisms that are not yet
understood (73).
Intriguingly, T6SSs may also be effective tools for gene acquisition via HGT. In V.
cholerae, the T6SS is co-regulated with competence genes by the regulator TfoX, and
transformation events are dependent upon the presence of an active T6SS (77). Borgeaud et
al. (2015) suggest that following activation of TfoX, both competence and T6SS systems are
expressed and assembled. After T6SS-mediated lysis of neighboring cells, DNA is released
to the extracellular space, where it can then transform the competent bacterium (77).
CONCLUDING REMARKS
Bacterial pathogens within the human host are exposed to a vast variety of different
selective pressures which shape bacterial genomes and drive the evolution of novel
virulence factors. Concomitantly, human genomes also evolve as a result of these
interactions, leading to a genetic ‘arms race’ between pathogens and their hosts. In bacteria,
horizontal gene transfer (HGT) can enhance this process by allowing for the rapid
dissemination of potentially beneficial alleles across strains or even species.
LITERATURE CITED
1. Toft C, Andersson SG. Evolutionary microbial genomics: insights into bacterial host adaptation.
Nature reviews Genetics. 2010; 11(7):465–75.
2. Dawkins R, Krebs JR. Arms races between and within species. Proceedings of the Royal Society of
London Series B, Biological sciences. 1979; 205(1161):489–511.
3. Woolhouse ME, Webster JP, Domingo E, Charlesworth B, Levin BR. Biological and biomedical
implications of the co-evolution of pathogens and their hosts. Nature genetics. 2002; 32(4):569–77.
[PubMed: 12457190]
4. Taubenberger JK, Kash JC. Influenza virus evolution, host adaptation, and pandemic formation.
Cell host & microbe. 2010; 7(6):440–51. [PubMed: 20542248]
5. Mideo N. Parasite adaptations to within-host competition. Trends in parasitology. 2009; 25(6):261–
8. [PubMed: 19409846]
Bliven and Maurelli Page 8
Microbiol Spectr. Author manuscript; available in PMC 2016 March 23.
Author Manuscript Author Manuscript Author Manuscript Author Manuscript
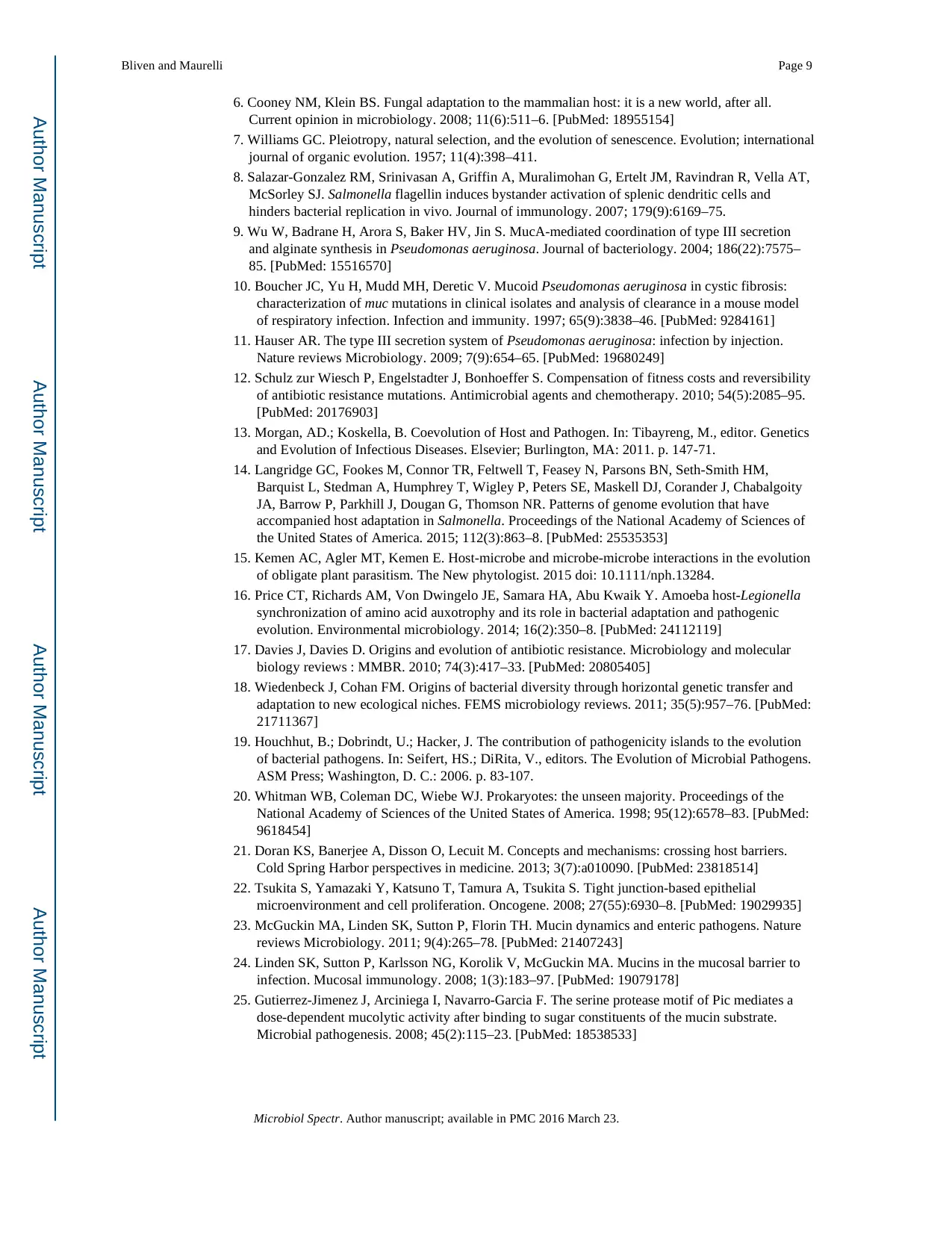
6. Cooney NM, Klein BS. Fungal adaptation to the mammalian host: it is a new world, after all.
Current opinion in microbiology. 2008; 11(6):511–6. [PubMed: 18955154]
7. Williams GC. Pleiotropy, natural selection, and the evolution of senescence. Evolution; international
journal of organic evolution. 1957; 11(4):398–411.
8. Salazar-Gonzalez RM, Srinivasan A, Griffin A, Muralimohan G, Ertelt JM, Ravindran R, Vella AT,
McSorley SJ. Salmonella flagellin induces bystander activation of splenic dendritic cells and
hinders bacterial replication in vivo. Journal of immunology. 2007; 179(9):6169–75.
9. Wu W, Badrane H, Arora S, Baker HV, Jin S. MucA-mediated coordination of type III secretion
and alginate synthesis in Pseudomonas aeruginosa. Journal of bacteriology. 2004; 186(22):7575–
85. [PubMed: 15516570]
10. Boucher JC, Yu H, Mudd MH, Deretic V. Mucoid Pseudomonas aeruginosa in cystic fibrosis:
characterization of muc mutations in clinical isolates and analysis of clearance in a mouse model
of respiratory infection. Infection and immunity. 1997; 65(9):3838–46. [PubMed: 9284161]
11. Hauser AR. The type III secretion system of Pseudomonas aeruginosa: infection by injection.
Nature reviews Microbiology. 2009; 7(9):654–65. [PubMed: 19680249]
12. Schulz zur Wiesch P, Engelstadter J, Bonhoeffer S. Compensation of fitness costs and reversibility
of antibiotic resistance mutations. Antimicrobial agents and chemotherapy. 2010; 54(5):2085–95.
[PubMed: 20176903]
13. Morgan, AD.; Koskella, B. Coevolution of Host and Pathogen. In: Tibayreng, M., editor. Genetics
and Evolution of Infectious Diseases. Elsevier; Burlington, MA: 2011. p. 147-71.
14. Langridge GC, Fookes M, Connor TR, Feltwell T, Feasey N, Parsons BN, Seth-Smith HM,
Barquist L, Stedman A, Humphrey T, Wigley P, Peters SE, Maskell DJ, Corander J, Chabalgoity
JA, Barrow P, Parkhill J, Dougan G, Thomson NR. Patterns of genome evolution that have
accompanied host adaptation in Salmonella. Proceedings of the National Academy of Sciences of
the United States of America. 2015; 112(3):863–8. [PubMed: 25535353]
15. Kemen AC, Agler MT, Kemen E. Host-microbe and microbe-microbe interactions in the evolution
of obligate plant parasitism. The New phytologist. 2015 doi: 10.1111/nph.13284.
16. Price CT, Richards AM, Von Dwingelo JE, Samara HA, Abu Kwaik Y. Amoeba host-Legionella
synchronization of amino acid auxotrophy and its role in bacterial adaptation and pathogenic
evolution. Environmental microbiology. 2014; 16(2):350–8. [PubMed: 24112119]
17. Davies J, Davies D. Origins and evolution of antibiotic resistance. Microbiology and molecular
biology reviews : MMBR. 2010; 74(3):417–33. [PubMed: 20805405]
18. Wiedenbeck J, Cohan FM. Origins of bacterial diversity through horizontal genetic transfer and
adaptation to new ecological niches. FEMS microbiology reviews. 2011; 35(5):957–76. [PubMed:
21711367]
19. Houchhut, B.; Dobrindt, U.; Hacker, J. The contribution of pathogenicity islands to the evolution
of bacterial pathogens. In: Seifert, HS.; DiRita, V., editors. The Evolution of Microbial Pathogens.
ASM Press; Washington, D. C.: 2006. p. 83-107.
20. Whitman WB, Coleman DC, Wiebe WJ. Prokaryotes: the unseen majority. Proceedings of the
National Academy of Sciences of the United States of America. 1998; 95(12):6578–83. [PubMed:
9618454]
21. Doran KS, Banerjee A, Disson O, Lecuit M. Concepts and mechanisms: crossing host barriers.
Cold Spring Harbor perspectives in medicine. 2013; 3(7):a010090. [PubMed: 23818514]
22. Tsukita S, Yamazaki Y, Katsuno T, Tamura A, Tsukita S. Tight junction-based epithelial
microenvironment and cell proliferation. Oncogene. 2008; 27(55):6930–8. [PubMed: 19029935]
23. McGuckin MA, Linden SK, Sutton P, Florin TH. Mucin dynamics and enteric pathogens. Nature
reviews Microbiology. 2011; 9(4):265–78. [PubMed: 21407243]
24. Linden SK, Sutton P, Karlsson NG, Korolik V, McGuckin MA. Mucins in the mucosal barrier to
infection. Mucosal immunology. 2008; 1(3):183–97. [PubMed: 19079178]
25. Gutierrez-Jimenez J, Arciniega I, Navarro-Garcia F. The serine protease motif of Pic mediates a
dose-dependent mucolytic activity after binding to sugar constituents of the mucin substrate.
Microbial pathogenesis. 2008; 45(2):115–23. [PubMed: 18538533]
Bliven and Maurelli Page 9
Microbiol Spectr. Author manuscript; available in PMC 2016 March 23.
Author Manuscript Author Manuscript Author Manuscript Author Manuscript
Current opinion in microbiology. 2008; 11(6):511–6. [PubMed: 18955154]
7. Williams GC. Pleiotropy, natural selection, and the evolution of senescence. Evolution; international
journal of organic evolution. 1957; 11(4):398–411.
8. Salazar-Gonzalez RM, Srinivasan A, Griffin A, Muralimohan G, Ertelt JM, Ravindran R, Vella AT,
McSorley SJ. Salmonella flagellin induces bystander activation of splenic dendritic cells and
hinders bacterial replication in vivo. Journal of immunology. 2007; 179(9):6169–75.
9. Wu W, Badrane H, Arora S, Baker HV, Jin S. MucA-mediated coordination of type III secretion
and alginate synthesis in Pseudomonas aeruginosa. Journal of bacteriology. 2004; 186(22):7575–
85. [PubMed: 15516570]
10. Boucher JC, Yu H, Mudd MH, Deretic V. Mucoid Pseudomonas aeruginosa in cystic fibrosis:
characterization of muc mutations in clinical isolates and analysis of clearance in a mouse model
of respiratory infection. Infection and immunity. 1997; 65(9):3838–46. [PubMed: 9284161]
11. Hauser AR. The type III secretion system of Pseudomonas aeruginosa: infection by injection.
Nature reviews Microbiology. 2009; 7(9):654–65. [PubMed: 19680249]
12. Schulz zur Wiesch P, Engelstadter J, Bonhoeffer S. Compensation of fitness costs and reversibility
of antibiotic resistance mutations. Antimicrobial agents and chemotherapy. 2010; 54(5):2085–95.
[PubMed: 20176903]
13. Morgan, AD.; Koskella, B. Coevolution of Host and Pathogen. In: Tibayreng, M., editor. Genetics
and Evolution of Infectious Diseases. Elsevier; Burlington, MA: 2011. p. 147-71.
14. Langridge GC, Fookes M, Connor TR, Feltwell T, Feasey N, Parsons BN, Seth-Smith HM,
Barquist L, Stedman A, Humphrey T, Wigley P, Peters SE, Maskell DJ, Corander J, Chabalgoity
JA, Barrow P, Parkhill J, Dougan G, Thomson NR. Patterns of genome evolution that have
accompanied host adaptation in Salmonella. Proceedings of the National Academy of Sciences of
the United States of America. 2015; 112(3):863–8. [PubMed: 25535353]
15. Kemen AC, Agler MT, Kemen E. Host-microbe and microbe-microbe interactions in the evolution
of obligate plant parasitism. The New phytologist. 2015 doi: 10.1111/nph.13284.
16. Price CT, Richards AM, Von Dwingelo JE, Samara HA, Abu Kwaik Y. Amoeba host-Legionella
synchronization of amino acid auxotrophy and its role in bacterial adaptation and pathogenic
evolution. Environmental microbiology. 2014; 16(2):350–8. [PubMed: 24112119]
17. Davies J, Davies D. Origins and evolution of antibiotic resistance. Microbiology and molecular
biology reviews : MMBR. 2010; 74(3):417–33. [PubMed: 20805405]
18. Wiedenbeck J, Cohan FM. Origins of bacterial diversity through horizontal genetic transfer and
adaptation to new ecological niches. FEMS microbiology reviews. 2011; 35(5):957–76. [PubMed:
21711367]
19. Houchhut, B.; Dobrindt, U.; Hacker, J. The contribution of pathogenicity islands to the evolution
of bacterial pathogens. In: Seifert, HS.; DiRita, V., editors. The Evolution of Microbial Pathogens.
ASM Press; Washington, D. C.: 2006. p. 83-107.
20. Whitman WB, Coleman DC, Wiebe WJ. Prokaryotes: the unseen majority. Proceedings of the
National Academy of Sciences of the United States of America. 1998; 95(12):6578–83. [PubMed:
9618454]
21. Doran KS, Banerjee A, Disson O, Lecuit M. Concepts and mechanisms: crossing host barriers.
Cold Spring Harbor perspectives in medicine. 2013; 3(7):a010090. [PubMed: 23818514]
22. Tsukita S, Yamazaki Y, Katsuno T, Tamura A, Tsukita S. Tight junction-based epithelial
microenvironment and cell proliferation. Oncogene. 2008; 27(55):6930–8. [PubMed: 19029935]
23. McGuckin MA, Linden SK, Sutton P, Florin TH. Mucin dynamics and enteric pathogens. Nature
reviews Microbiology. 2011; 9(4):265–78. [PubMed: 21407243]
24. Linden SK, Sutton P, Karlsson NG, Korolik V, McGuckin MA. Mucins in the mucosal barrier to
infection. Mucosal immunology. 2008; 1(3):183–97. [PubMed: 19079178]
25. Gutierrez-Jimenez J, Arciniega I, Navarro-Garcia F. The serine protease motif of Pic mediates a
dose-dependent mucolytic activity after binding to sugar constituents of the mucin substrate.
Microbial pathogenesis. 2008; 45(2):115–23. [PubMed: 18538533]
Bliven and Maurelli Page 9
Microbiol Spectr. Author manuscript; available in PMC 2016 March 23.
Author Manuscript Author Manuscript Author Manuscript Author Manuscript
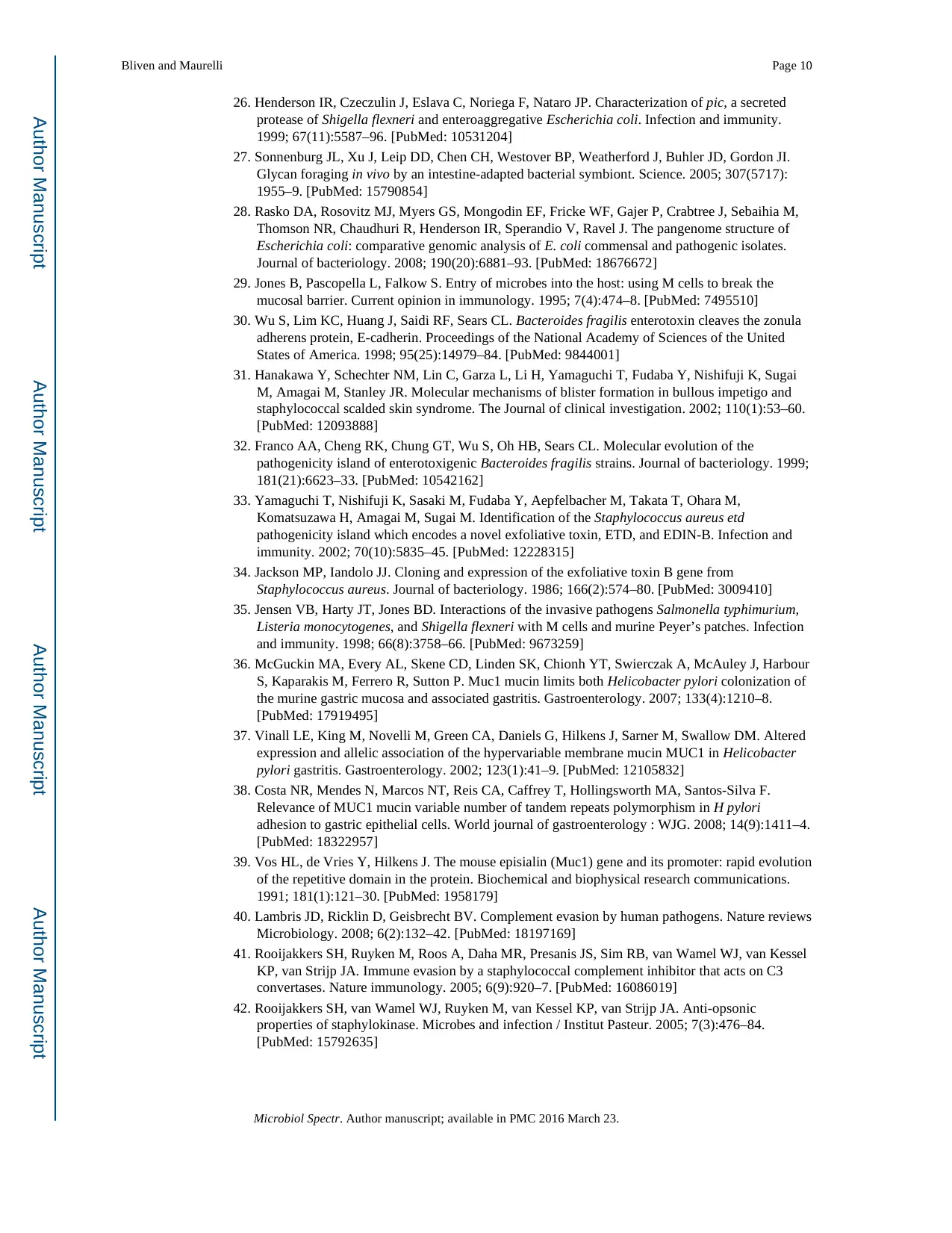
26. Henderson IR, Czeczulin J, Eslava C, Noriega F, Nataro JP. Characterization of pic, a secreted
protease of Shigella flexneri and enteroaggregative Escherichia coli. Infection and immunity.
1999; 67(11):5587–96. [PubMed: 10531204]
27. Sonnenburg JL, Xu J, Leip DD, Chen CH, Westover BP, Weatherford J, Buhler JD, Gordon JI.
Glycan foraging in vivo by an intestine-adapted bacterial symbiont. Science. 2005; 307(5717):
1955–9. [PubMed: 15790854]
28. Rasko DA, Rosovitz MJ, Myers GS, Mongodin EF, Fricke WF, Gajer P, Crabtree J, Sebaihia M,
Thomson NR, Chaudhuri R, Henderson IR, Sperandio V, Ravel J. The pangenome structure of
Escherichia coli: comparative genomic analysis of E. coli commensal and pathogenic isolates.
Journal of bacteriology. 2008; 190(20):6881–93. [PubMed: 18676672]
29. Jones B, Pascopella L, Falkow S. Entry of microbes into the host: using M cells to break the
mucosal barrier. Current opinion in immunology. 1995; 7(4):474–8. [PubMed: 7495510]
30. Wu S, Lim KC, Huang J, Saidi RF, Sears CL. Bacteroides fragilis enterotoxin cleaves the zonula
adherens protein, E-cadherin. Proceedings of the National Academy of Sciences of the United
States of America. 1998; 95(25):14979–84. [PubMed: 9844001]
31. Hanakawa Y, Schechter NM, Lin C, Garza L, Li H, Yamaguchi T, Fudaba Y, Nishifuji K, Sugai
M, Amagai M, Stanley JR. Molecular mechanisms of blister formation in bullous impetigo and
staphylococcal scalded skin syndrome. The Journal of clinical investigation. 2002; 110(1):53–60.
[PubMed: 12093888]
32. Franco AA, Cheng RK, Chung GT, Wu S, Oh HB, Sears CL. Molecular evolution of the
pathogenicity island of enterotoxigenic Bacteroides fragilis strains. Journal of bacteriology. 1999;
181(21):6623–33. [PubMed: 10542162]
33. Yamaguchi T, Nishifuji K, Sasaki M, Fudaba Y, Aepfelbacher M, Takata T, Ohara M,
Komatsuzawa H, Amagai M, Sugai M. Identification of the Staphylococcus aureus etd
pathogenicity island which encodes a novel exfoliative toxin, ETD, and EDIN-B. Infection and
immunity. 2002; 70(10):5835–45. [PubMed: 12228315]
34. Jackson MP, Iandolo JJ. Cloning and expression of the exfoliative toxin B gene from
Staphylococcus aureus. Journal of bacteriology. 1986; 166(2):574–80. [PubMed: 3009410]
35. Jensen VB, Harty JT, Jones BD. Interactions of the invasive pathogens Salmonella typhimurium,
Listeria monocytogenes, and Shigella flexneri with M cells and murine Peyer’s patches. Infection
and immunity. 1998; 66(8):3758–66. [PubMed: 9673259]
36. McGuckin MA, Every AL, Skene CD, Linden SK, Chionh YT, Swierczak A, McAuley J, Harbour
S, Kaparakis M, Ferrero R, Sutton P. Muc1 mucin limits both Helicobacter pylori colonization of
the murine gastric mucosa and associated gastritis. Gastroenterology. 2007; 133(4):1210–8.
[PubMed: 17919495]
37. Vinall LE, King M, Novelli M, Green CA, Daniels G, Hilkens J, Sarner M, Swallow DM. Altered
expression and allelic association of the hypervariable membrane mucin MUC1 in Helicobacter
pylori gastritis. Gastroenterology. 2002; 123(1):41–9. [PubMed: 12105832]
38. Costa NR, Mendes N, Marcos NT, Reis CA, Caffrey T, Hollingsworth MA, Santos-Silva F.
Relevance of MUC1 mucin variable number of tandem repeats polymorphism in H pylori
adhesion to gastric epithelial cells. World journal of gastroenterology : WJG. 2008; 14(9):1411–4.
[PubMed: 18322957]
39. Vos HL, de Vries Y, Hilkens J. The mouse episialin (Muc1) gene and its promoter: rapid evolution
of the repetitive domain in the protein. Biochemical and biophysical research communications.
1991; 181(1):121–30. [PubMed: 1958179]
40. Lambris JD, Ricklin D, Geisbrecht BV. Complement evasion by human pathogens. Nature reviews
Microbiology. 2008; 6(2):132–42. [PubMed: 18197169]
41. Rooijakkers SH, Ruyken M, Roos A, Daha MR, Presanis JS, Sim RB, van Wamel WJ, van Kessel
KP, van Strijp JA. Immune evasion by a staphylococcal complement inhibitor that acts on C3
convertases. Nature immunology. 2005; 6(9):920–7. [PubMed: 16086019]
42. Rooijakkers SH, van Wamel WJ, Ruyken M, van Kessel KP, van Strijp JA. Anti-opsonic
properties of staphylokinase. Microbes and infection / Institut Pasteur. 2005; 7(3):476–84.
[PubMed: 15792635]
Bliven and Maurelli Page 10
Microbiol Spectr. Author manuscript; available in PMC 2016 March 23.
Author Manuscript Author Manuscript Author Manuscript Author Manuscript
protease of Shigella flexneri and enteroaggregative Escherichia coli. Infection and immunity.
1999; 67(11):5587–96. [PubMed: 10531204]
27. Sonnenburg JL, Xu J, Leip DD, Chen CH, Westover BP, Weatherford J, Buhler JD, Gordon JI.
Glycan foraging in vivo by an intestine-adapted bacterial symbiont. Science. 2005; 307(5717):
1955–9. [PubMed: 15790854]
28. Rasko DA, Rosovitz MJ, Myers GS, Mongodin EF, Fricke WF, Gajer P, Crabtree J, Sebaihia M,
Thomson NR, Chaudhuri R, Henderson IR, Sperandio V, Ravel J. The pangenome structure of
Escherichia coli: comparative genomic analysis of E. coli commensal and pathogenic isolates.
Journal of bacteriology. 2008; 190(20):6881–93. [PubMed: 18676672]
29. Jones B, Pascopella L, Falkow S. Entry of microbes into the host: using M cells to break the
mucosal barrier. Current opinion in immunology. 1995; 7(4):474–8. [PubMed: 7495510]
30. Wu S, Lim KC, Huang J, Saidi RF, Sears CL. Bacteroides fragilis enterotoxin cleaves the zonula
adherens protein, E-cadherin. Proceedings of the National Academy of Sciences of the United
States of America. 1998; 95(25):14979–84. [PubMed: 9844001]
31. Hanakawa Y, Schechter NM, Lin C, Garza L, Li H, Yamaguchi T, Fudaba Y, Nishifuji K, Sugai
M, Amagai M, Stanley JR. Molecular mechanisms of blister formation in bullous impetigo and
staphylococcal scalded skin syndrome. The Journal of clinical investigation. 2002; 110(1):53–60.
[PubMed: 12093888]
32. Franco AA, Cheng RK, Chung GT, Wu S, Oh HB, Sears CL. Molecular evolution of the
pathogenicity island of enterotoxigenic Bacteroides fragilis strains. Journal of bacteriology. 1999;
181(21):6623–33. [PubMed: 10542162]
33. Yamaguchi T, Nishifuji K, Sasaki M, Fudaba Y, Aepfelbacher M, Takata T, Ohara M,
Komatsuzawa H, Amagai M, Sugai M. Identification of the Staphylococcus aureus etd
pathogenicity island which encodes a novel exfoliative toxin, ETD, and EDIN-B. Infection and
immunity. 2002; 70(10):5835–45. [PubMed: 12228315]
34. Jackson MP, Iandolo JJ. Cloning and expression of the exfoliative toxin B gene from
Staphylococcus aureus. Journal of bacteriology. 1986; 166(2):574–80. [PubMed: 3009410]
35. Jensen VB, Harty JT, Jones BD. Interactions of the invasive pathogens Salmonella typhimurium,
Listeria monocytogenes, and Shigella flexneri with M cells and murine Peyer’s patches. Infection
and immunity. 1998; 66(8):3758–66. [PubMed: 9673259]
36. McGuckin MA, Every AL, Skene CD, Linden SK, Chionh YT, Swierczak A, McAuley J, Harbour
S, Kaparakis M, Ferrero R, Sutton P. Muc1 mucin limits both Helicobacter pylori colonization of
the murine gastric mucosa and associated gastritis. Gastroenterology. 2007; 133(4):1210–8.
[PubMed: 17919495]
37. Vinall LE, King M, Novelli M, Green CA, Daniels G, Hilkens J, Sarner M, Swallow DM. Altered
expression and allelic association of the hypervariable membrane mucin MUC1 in Helicobacter
pylori gastritis. Gastroenterology. 2002; 123(1):41–9. [PubMed: 12105832]
38. Costa NR, Mendes N, Marcos NT, Reis CA, Caffrey T, Hollingsworth MA, Santos-Silva F.
Relevance of MUC1 mucin variable number of tandem repeats polymorphism in H pylori
adhesion to gastric epithelial cells. World journal of gastroenterology : WJG. 2008; 14(9):1411–4.
[PubMed: 18322957]
39. Vos HL, de Vries Y, Hilkens J. The mouse episialin (Muc1) gene and its promoter: rapid evolution
of the repetitive domain in the protein. Biochemical and biophysical research communications.
1991; 181(1):121–30. [PubMed: 1958179]
40. Lambris JD, Ricklin D, Geisbrecht BV. Complement evasion by human pathogens. Nature reviews
Microbiology. 2008; 6(2):132–42. [PubMed: 18197169]
41. Rooijakkers SH, Ruyken M, Roos A, Daha MR, Presanis JS, Sim RB, van Wamel WJ, van Kessel
KP, van Strijp JA. Immune evasion by a staphylococcal complement inhibitor that acts on C3
convertases. Nature immunology. 2005; 6(9):920–7. [PubMed: 16086019]
42. Rooijakkers SH, van Wamel WJ, Ruyken M, van Kessel KP, van Strijp JA. Anti-opsonic
properties of staphylokinase. Microbes and infection / Institut Pasteur. 2005; 7(3):476–84.
[PubMed: 15792635]
Bliven and Maurelli Page 10
Microbiol Spectr. Author manuscript; available in PMC 2016 March 23.
Author Manuscript Author Manuscript Author Manuscript Author Manuscript
Secure Best Marks with AI Grader
Need help grading? Try our AI Grader for instant feedback on your assignments.
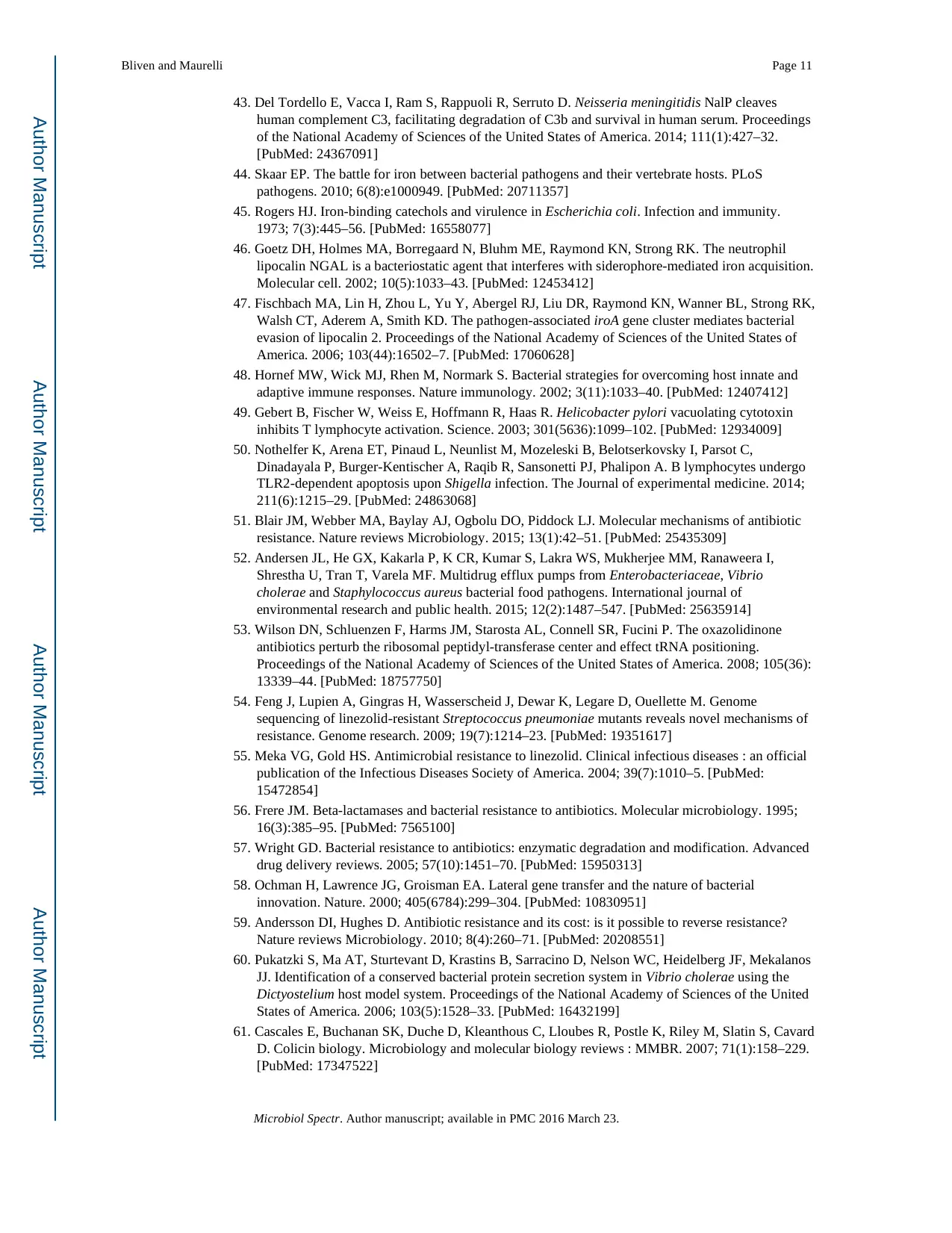
43. Del Tordello E, Vacca I, Ram S, Rappuoli R, Serruto D. Neisseria meningitidis NalP cleaves
human complement C3, facilitating degradation of C3b and survival in human serum. Proceedings
of the National Academy of Sciences of the United States of America. 2014; 111(1):427–32.
[PubMed: 24367091]
44. Skaar EP. The battle for iron between bacterial pathogens and their vertebrate hosts. PLoS
pathogens. 2010; 6(8):e1000949. [PubMed: 20711357]
45. Rogers HJ. Iron-binding catechols and virulence in Escherichia coli. Infection and immunity.
1973; 7(3):445–56. [PubMed: 16558077]
46. Goetz DH, Holmes MA, Borregaard N, Bluhm ME, Raymond KN, Strong RK. The neutrophil
lipocalin NGAL is a bacteriostatic agent that interferes with siderophore-mediated iron acquisition.
Molecular cell. 2002; 10(5):1033–43. [PubMed: 12453412]
47. Fischbach MA, Lin H, Zhou L, Yu Y, Abergel RJ, Liu DR, Raymond KN, Wanner BL, Strong RK,
Walsh CT, Aderem A, Smith KD. The pathogen-associated iroA gene cluster mediates bacterial
evasion of lipocalin 2. Proceedings of the National Academy of Sciences of the United States of
America. 2006; 103(44):16502–7. [PubMed: 17060628]
48. Hornef MW, Wick MJ, Rhen M, Normark S. Bacterial strategies for overcoming host innate and
adaptive immune responses. Nature immunology. 2002; 3(11):1033–40. [PubMed: 12407412]
49. Gebert B, Fischer W, Weiss E, Hoffmann R, Haas R. Helicobacter pylori vacuolating cytotoxin
inhibits T lymphocyte activation. Science. 2003; 301(5636):1099–102. [PubMed: 12934009]
50. Nothelfer K, Arena ET, Pinaud L, Neunlist M, Mozeleski B, Belotserkovsky I, Parsot C,
Dinadayala P, Burger-Kentischer A, Raqib R, Sansonetti PJ, Phalipon A. B lymphocytes undergo
TLR2-dependent apoptosis upon Shigella infection. The Journal of experimental medicine. 2014;
211(6):1215–29. [PubMed: 24863068]
51. Blair JM, Webber MA, Baylay AJ, Ogbolu DO, Piddock LJ. Molecular mechanisms of antibiotic
resistance. Nature reviews Microbiology. 2015; 13(1):42–51. [PubMed: 25435309]
52. Andersen JL, He GX, Kakarla P, K CR, Kumar S, Lakra WS, Mukherjee MM, Ranaweera I,
Shrestha U, Tran T, Varela MF. Multidrug efflux pumps from Enterobacteriaceae, Vibrio
cholerae and Staphylococcus aureus bacterial food pathogens. International journal of
environmental research and public health. 2015; 12(2):1487–547. [PubMed: 25635914]
53. Wilson DN, Schluenzen F, Harms JM, Starosta AL, Connell SR, Fucini P. The oxazolidinone
antibiotics perturb the ribosomal peptidyl-transferase center and effect tRNA positioning.
Proceedings of the National Academy of Sciences of the United States of America. 2008; 105(36):
13339–44. [PubMed: 18757750]
54. Feng J, Lupien A, Gingras H, Wasserscheid J, Dewar K, Legare D, Ouellette M. Genome
sequencing of linezolid-resistant Streptococcus pneumoniae mutants reveals novel mechanisms of
resistance. Genome research. 2009; 19(7):1214–23. [PubMed: 19351617]
55. Meka VG, Gold HS. Antimicrobial resistance to linezolid. Clinical infectious diseases : an official
publication of the Infectious Diseases Society of America. 2004; 39(7):1010–5. [PubMed:
15472854]
56. Frere JM. Beta-lactamases and bacterial resistance to antibiotics. Molecular microbiology. 1995;
16(3):385–95. [PubMed: 7565100]
57. Wright GD. Bacterial resistance to antibiotics: enzymatic degradation and modification. Advanced
drug delivery reviews. 2005; 57(10):1451–70. [PubMed: 15950313]
58. Ochman H, Lawrence JG, Groisman EA. Lateral gene transfer and the nature of bacterial
innovation. Nature. 2000; 405(6784):299–304. [PubMed: 10830951]
59. Andersson DI, Hughes D. Antibiotic resistance and its cost: is it possible to reverse resistance?
Nature reviews Microbiology. 2010; 8(4):260–71. [PubMed: 20208551]
60. Pukatzki S, Ma AT, Sturtevant D, Krastins B, Sarracino D, Nelson WC, Heidelberg JF, Mekalanos
JJ. Identification of a conserved bacterial protein secretion system in Vibrio cholerae using the
Dictyostelium host model system. Proceedings of the National Academy of Sciences of the United
States of America. 2006; 103(5):1528–33. [PubMed: 16432199]
61. Cascales E, Buchanan SK, Duche D, Kleanthous C, Lloubes R, Postle K, Riley M, Slatin S, Cavard
D. Colicin biology. Microbiology and molecular biology reviews : MMBR. 2007; 71(1):158–229.
[PubMed: 17347522]
Bliven and Maurelli Page 11
Microbiol Spectr. Author manuscript; available in PMC 2016 March 23.
Author Manuscript Author Manuscript Author Manuscript Author Manuscript
human complement C3, facilitating degradation of C3b and survival in human serum. Proceedings
of the National Academy of Sciences of the United States of America. 2014; 111(1):427–32.
[PubMed: 24367091]
44. Skaar EP. The battle for iron between bacterial pathogens and their vertebrate hosts. PLoS
pathogens. 2010; 6(8):e1000949. [PubMed: 20711357]
45. Rogers HJ. Iron-binding catechols and virulence in Escherichia coli. Infection and immunity.
1973; 7(3):445–56. [PubMed: 16558077]
46. Goetz DH, Holmes MA, Borregaard N, Bluhm ME, Raymond KN, Strong RK. The neutrophil
lipocalin NGAL is a bacteriostatic agent that interferes with siderophore-mediated iron acquisition.
Molecular cell. 2002; 10(5):1033–43. [PubMed: 12453412]
47. Fischbach MA, Lin H, Zhou L, Yu Y, Abergel RJ, Liu DR, Raymond KN, Wanner BL, Strong RK,
Walsh CT, Aderem A, Smith KD. The pathogen-associated iroA gene cluster mediates bacterial
evasion of lipocalin 2. Proceedings of the National Academy of Sciences of the United States of
America. 2006; 103(44):16502–7. [PubMed: 17060628]
48. Hornef MW, Wick MJ, Rhen M, Normark S. Bacterial strategies for overcoming host innate and
adaptive immune responses. Nature immunology. 2002; 3(11):1033–40. [PubMed: 12407412]
49. Gebert B, Fischer W, Weiss E, Hoffmann R, Haas R. Helicobacter pylori vacuolating cytotoxin
inhibits T lymphocyte activation. Science. 2003; 301(5636):1099–102. [PubMed: 12934009]
50. Nothelfer K, Arena ET, Pinaud L, Neunlist M, Mozeleski B, Belotserkovsky I, Parsot C,
Dinadayala P, Burger-Kentischer A, Raqib R, Sansonetti PJ, Phalipon A. B lymphocytes undergo
TLR2-dependent apoptosis upon Shigella infection. The Journal of experimental medicine. 2014;
211(6):1215–29. [PubMed: 24863068]
51. Blair JM, Webber MA, Baylay AJ, Ogbolu DO, Piddock LJ. Molecular mechanisms of antibiotic
resistance. Nature reviews Microbiology. 2015; 13(1):42–51. [PubMed: 25435309]
52. Andersen JL, He GX, Kakarla P, K CR, Kumar S, Lakra WS, Mukherjee MM, Ranaweera I,
Shrestha U, Tran T, Varela MF. Multidrug efflux pumps from Enterobacteriaceae, Vibrio
cholerae and Staphylococcus aureus bacterial food pathogens. International journal of
environmental research and public health. 2015; 12(2):1487–547. [PubMed: 25635914]
53. Wilson DN, Schluenzen F, Harms JM, Starosta AL, Connell SR, Fucini P. The oxazolidinone
antibiotics perturb the ribosomal peptidyl-transferase center and effect tRNA positioning.
Proceedings of the National Academy of Sciences of the United States of America. 2008; 105(36):
13339–44. [PubMed: 18757750]
54. Feng J, Lupien A, Gingras H, Wasserscheid J, Dewar K, Legare D, Ouellette M. Genome
sequencing of linezolid-resistant Streptococcus pneumoniae mutants reveals novel mechanisms of
resistance. Genome research. 2009; 19(7):1214–23. [PubMed: 19351617]
55. Meka VG, Gold HS. Antimicrobial resistance to linezolid. Clinical infectious diseases : an official
publication of the Infectious Diseases Society of America. 2004; 39(7):1010–5. [PubMed:
15472854]
56. Frere JM. Beta-lactamases and bacterial resistance to antibiotics. Molecular microbiology. 1995;
16(3):385–95. [PubMed: 7565100]
57. Wright GD. Bacterial resistance to antibiotics: enzymatic degradation and modification. Advanced
drug delivery reviews. 2005; 57(10):1451–70. [PubMed: 15950313]
58. Ochman H, Lawrence JG, Groisman EA. Lateral gene transfer and the nature of bacterial
innovation. Nature. 2000; 405(6784):299–304. [PubMed: 10830951]
59. Andersson DI, Hughes D. Antibiotic resistance and its cost: is it possible to reverse resistance?
Nature reviews Microbiology. 2010; 8(4):260–71. [PubMed: 20208551]
60. Pukatzki S, Ma AT, Sturtevant D, Krastins B, Sarracino D, Nelson WC, Heidelberg JF, Mekalanos
JJ. Identification of a conserved bacterial protein secretion system in Vibrio cholerae using the
Dictyostelium host model system. Proceedings of the National Academy of Sciences of the United
States of America. 2006; 103(5):1528–33. [PubMed: 16432199]
61. Cascales E, Buchanan SK, Duche D, Kleanthous C, Lloubes R, Postle K, Riley M, Slatin S, Cavard
D. Colicin biology. Microbiology and molecular biology reviews : MMBR. 2007; 71(1):158–229.
[PubMed: 17347522]
Bliven and Maurelli Page 11
Microbiol Spectr. Author manuscript; available in PMC 2016 March 23.
Author Manuscript Author Manuscript Author Manuscript Author Manuscript
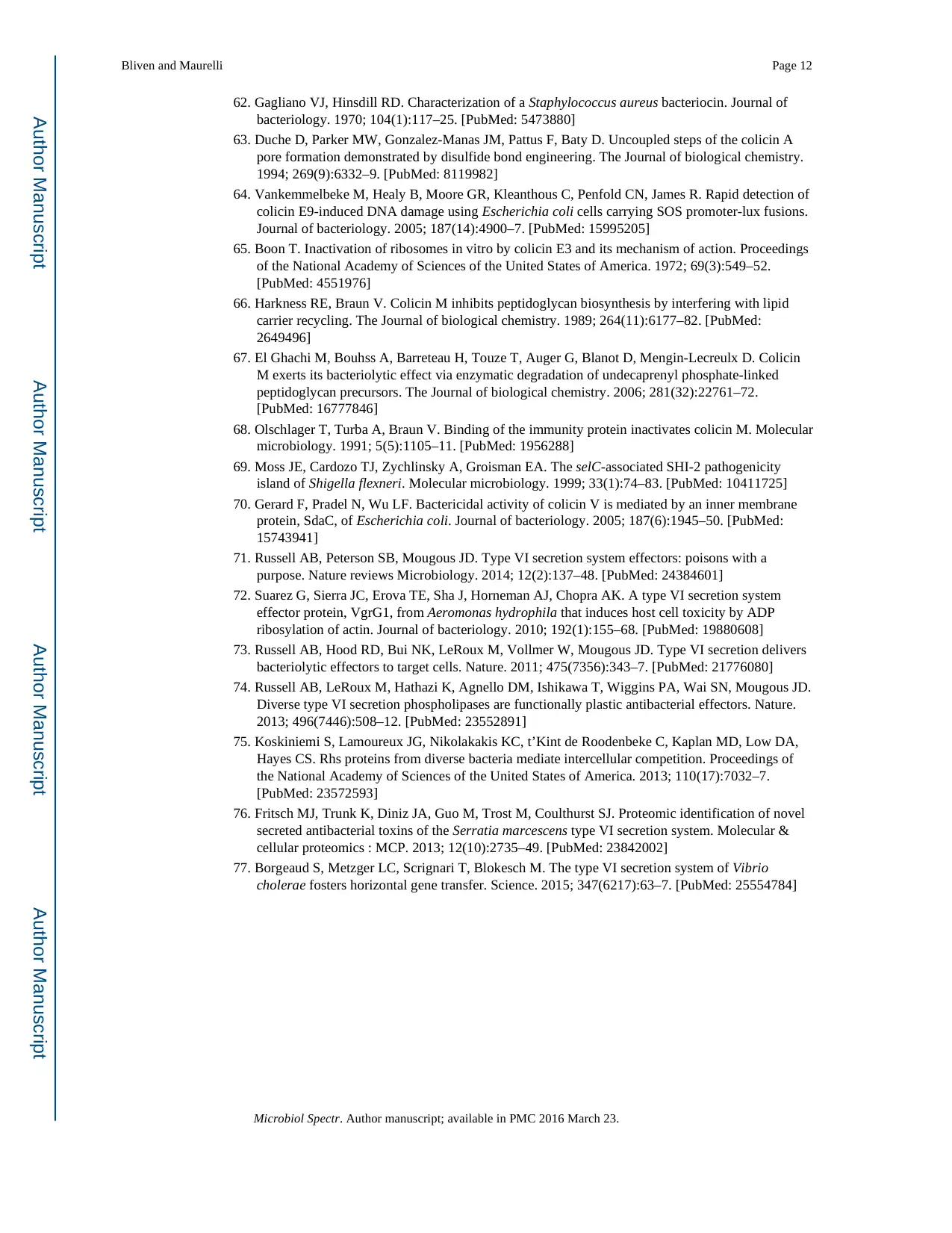
62. Gagliano VJ, Hinsdill RD. Characterization of a Staphylococcus aureus bacteriocin. Journal of
bacteriology. 1970; 104(1):117–25. [PubMed: 5473880]
63. Duche D, Parker MW, Gonzalez-Manas JM, Pattus F, Baty D. Uncoupled steps of the colicin A
pore formation demonstrated by disulfide bond engineering. The Journal of biological chemistry.
1994; 269(9):6332–9. [PubMed: 8119982]
64. Vankemmelbeke M, Healy B, Moore GR, Kleanthous C, Penfold CN, James R. Rapid detection of
colicin E9-induced DNA damage using Escherichia coli cells carrying SOS promoter-lux fusions.
Journal of bacteriology. 2005; 187(14):4900–7. [PubMed: 15995205]
65. Boon T. Inactivation of ribosomes in vitro by colicin E3 and its mechanism of action. Proceedings
of the National Academy of Sciences of the United States of America. 1972; 69(3):549–52.
[PubMed: 4551976]
66. Harkness RE, Braun V. Colicin M inhibits peptidoglycan biosynthesis by interfering with lipid
carrier recycling. The Journal of biological chemistry. 1989; 264(11):6177–82. [PubMed:
2649496]
67. El Ghachi M, Bouhss A, Barreteau H, Touze T, Auger G, Blanot D, Mengin-Lecreulx D. Colicin
M exerts its bacteriolytic effect via enzymatic degradation of undecaprenyl phosphate-linked
peptidoglycan precursors. The Journal of biological chemistry. 2006; 281(32):22761–72.
[PubMed: 16777846]
68. Olschlager T, Turba A, Braun V. Binding of the immunity protein inactivates colicin M. Molecular
microbiology. 1991; 5(5):1105–11. [PubMed: 1956288]
69. Moss JE, Cardozo TJ, Zychlinsky A, Groisman EA. The selC-associated SHI-2 pathogenicity
island of Shigella flexneri. Molecular microbiology. 1999; 33(1):74–83. [PubMed: 10411725]
70. Gerard F, Pradel N, Wu LF. Bactericidal activity of colicin V is mediated by an inner membrane
protein, SdaC, of Escherichia coli. Journal of bacteriology. 2005; 187(6):1945–50. [PubMed:
15743941]
71. Russell AB, Peterson SB, Mougous JD. Type VI secretion system effectors: poisons with a
purpose. Nature reviews Microbiology. 2014; 12(2):137–48. [PubMed: 24384601]
72. Suarez G, Sierra JC, Erova TE, Sha J, Horneman AJ, Chopra AK. A type VI secretion system
effector protein, VgrG1, from Aeromonas hydrophila that induces host cell toxicity by ADP
ribosylation of actin. Journal of bacteriology. 2010; 192(1):155–68. [PubMed: 19880608]
73. Russell AB, Hood RD, Bui NK, LeRoux M, Vollmer W, Mougous JD. Type VI secretion delivers
bacteriolytic effectors to target cells. Nature. 2011; 475(7356):343–7. [PubMed: 21776080]
74. Russell AB, LeRoux M, Hathazi K, Agnello DM, Ishikawa T, Wiggins PA, Wai SN, Mougous JD.
Diverse type VI secretion phospholipases are functionally plastic antibacterial effectors. Nature.
2013; 496(7446):508–12. [PubMed: 23552891]
75. Koskiniemi S, Lamoureux JG, Nikolakakis KC, t’Kint de Roodenbeke C, Kaplan MD, Low DA,
Hayes CS. Rhs proteins from diverse bacteria mediate intercellular competition. Proceedings of
the National Academy of Sciences of the United States of America. 2013; 110(17):7032–7.
[PubMed: 23572593]
76. Fritsch MJ, Trunk K, Diniz JA, Guo M, Trost M, Coulthurst SJ. Proteomic identification of novel
secreted antibacterial toxins of the Serratia marcescens type VI secretion system. Molecular &
cellular proteomics : MCP. 2013; 12(10):2735–49. [PubMed: 23842002]
77. Borgeaud S, Metzger LC, Scrignari T, Blokesch M. The type VI secretion system of Vibrio
cholerae fosters horizontal gene transfer. Science. 2015; 347(6217):63–7. [PubMed: 25554784]
Bliven and Maurelli Page 12
Microbiol Spectr. Author manuscript; available in PMC 2016 March 23.
Author Manuscript Author Manuscript Author Manuscript Author Manuscript
bacteriology. 1970; 104(1):117–25. [PubMed: 5473880]
63. Duche D, Parker MW, Gonzalez-Manas JM, Pattus F, Baty D. Uncoupled steps of the colicin A
pore formation demonstrated by disulfide bond engineering. The Journal of biological chemistry.
1994; 269(9):6332–9. [PubMed: 8119982]
64. Vankemmelbeke M, Healy B, Moore GR, Kleanthous C, Penfold CN, James R. Rapid detection of
colicin E9-induced DNA damage using Escherichia coli cells carrying SOS promoter-lux fusions.
Journal of bacteriology. 2005; 187(14):4900–7. [PubMed: 15995205]
65. Boon T. Inactivation of ribosomes in vitro by colicin E3 and its mechanism of action. Proceedings
of the National Academy of Sciences of the United States of America. 1972; 69(3):549–52.
[PubMed: 4551976]
66. Harkness RE, Braun V. Colicin M inhibits peptidoglycan biosynthesis by interfering with lipid
carrier recycling. The Journal of biological chemistry. 1989; 264(11):6177–82. [PubMed:
2649496]
67. El Ghachi M, Bouhss A, Barreteau H, Touze T, Auger G, Blanot D, Mengin-Lecreulx D. Colicin
M exerts its bacteriolytic effect via enzymatic degradation of undecaprenyl phosphate-linked
peptidoglycan precursors. The Journal of biological chemistry. 2006; 281(32):22761–72.
[PubMed: 16777846]
68. Olschlager T, Turba A, Braun V. Binding of the immunity protein inactivates colicin M. Molecular
microbiology. 1991; 5(5):1105–11. [PubMed: 1956288]
69. Moss JE, Cardozo TJ, Zychlinsky A, Groisman EA. The selC-associated SHI-2 pathogenicity
island of Shigella flexneri. Molecular microbiology. 1999; 33(1):74–83. [PubMed: 10411725]
70. Gerard F, Pradel N, Wu LF. Bactericidal activity of colicin V is mediated by an inner membrane
protein, SdaC, of Escherichia coli. Journal of bacteriology. 2005; 187(6):1945–50. [PubMed:
15743941]
71. Russell AB, Peterson SB, Mougous JD. Type VI secretion system effectors: poisons with a
purpose. Nature reviews Microbiology. 2014; 12(2):137–48. [PubMed: 24384601]
72. Suarez G, Sierra JC, Erova TE, Sha J, Horneman AJ, Chopra AK. A type VI secretion system
effector protein, VgrG1, from Aeromonas hydrophila that induces host cell toxicity by ADP
ribosylation of actin. Journal of bacteriology. 2010; 192(1):155–68. [PubMed: 19880608]
73. Russell AB, Hood RD, Bui NK, LeRoux M, Vollmer W, Mougous JD. Type VI secretion delivers
bacteriolytic effectors to target cells. Nature. 2011; 475(7356):343–7. [PubMed: 21776080]
74. Russell AB, LeRoux M, Hathazi K, Agnello DM, Ishikawa T, Wiggins PA, Wai SN, Mougous JD.
Diverse type VI secretion phospholipases are functionally plastic antibacterial effectors. Nature.
2013; 496(7446):508–12. [PubMed: 23552891]
75. Koskiniemi S, Lamoureux JG, Nikolakakis KC, t’Kint de Roodenbeke C, Kaplan MD, Low DA,
Hayes CS. Rhs proteins from diverse bacteria mediate intercellular competition. Proceedings of
the National Academy of Sciences of the United States of America. 2013; 110(17):7032–7.
[PubMed: 23572593]
76. Fritsch MJ, Trunk K, Diniz JA, Guo M, Trost M, Coulthurst SJ. Proteomic identification of novel
secreted antibacterial toxins of the Serratia marcescens type VI secretion system. Molecular &
cellular proteomics : MCP. 2013; 12(10):2735–49. [PubMed: 23842002]
77. Borgeaud S, Metzger LC, Scrignari T, Blokesch M. The type VI secretion system of Vibrio
cholerae fosters horizontal gene transfer. Science. 2015; 347(6217):63–7. [PubMed: 25554784]
Bliven and Maurelli Page 12
Microbiol Spectr. Author manuscript; available in PMC 2016 March 23.
Author Manuscript Author Manuscript Author Manuscript Author Manuscript
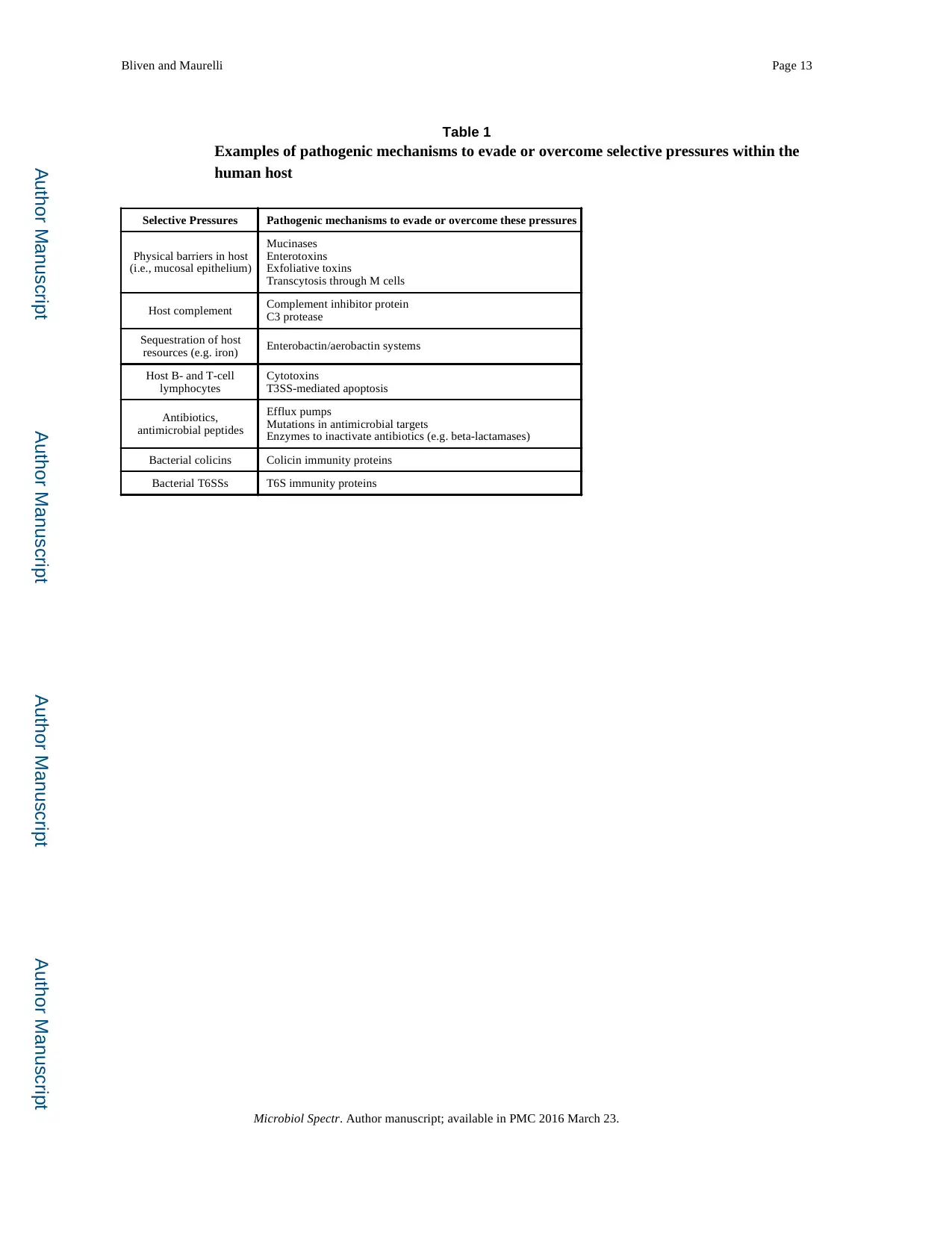
Author Manuscript Author Manuscript Author Manuscript Author Manuscript
Bliven and Maurelli Page 13
Table 1
Examples of pathogenic mechanisms to evade or overcome selective pressures within the
human host
Selective Pressures Pathogenic mechanisms to evade or overcome these pressures
Physical barriers in host
(i.e., mucosal epithelium)
Mucinases
Enterotoxins
Exfoliative toxins
Transcytosis through M cells
Host complement Complement inhibitor protein
C3 protease
Sequestration of host
resources (e.g. iron) Enterobactin/aerobactin systems
Host B- and T-cell
lymphocytes
Cytotoxins
T3SS-mediated apoptosis
Antibiotics,
antimicrobial peptides
Efflux pumps
Mutations in antimicrobial targets
Enzymes to inactivate antibiotics (e.g. beta-lactamases)
Bacterial colicins Colicin immunity proteins
Bacterial T6SSs T6S immunity proteins
Microbiol Spectr. Author manuscript; available in PMC 2016 March 23.
Bliven and Maurelli Page 13
Table 1
Examples of pathogenic mechanisms to evade or overcome selective pressures within the
human host
Selective Pressures Pathogenic mechanisms to evade or overcome these pressures
Physical barriers in host
(i.e., mucosal epithelium)
Mucinases
Enterotoxins
Exfoliative toxins
Transcytosis through M cells
Host complement Complement inhibitor protein
C3 protease
Sequestration of host
resources (e.g. iron) Enterobactin/aerobactin systems
Host B- and T-cell
lymphocytes
Cytotoxins
T3SS-mediated apoptosis
Antibiotics,
antimicrobial peptides
Efflux pumps
Mutations in antimicrobial targets
Enzymes to inactivate antibiotics (e.g. beta-lactamases)
Bacterial colicins Colicin immunity proteins
Bacterial T6SSs T6S immunity proteins
Microbiol Spectr. Author manuscript; available in PMC 2016 March 23.
1 out of 13
![[object Object]](/_next/image/?url=%2F_next%2Fstatic%2Fmedia%2Flogo.6d15ce61.png&w=640&q=75)
Your All-in-One AI-Powered Toolkit for Academic Success.
+13062052269
info@desklib.com
Available 24*7 on WhatsApp / Email
Unlock your academic potential
© 2024 | Zucol Services PVT LTD | All rights reserved.