Impact of Advanced Imaging Technology in Biology
VerifiedAdded on 2023/04/20
|14
|3686
|395
AI Summary
This paper explores the impact of advanced imaging technology in improving our understanding of biology. It discusses the advancements in imaging technology and the essentiality of these advancements in processing and visualization. The paper also highlights the impact of new tracers, probes, and sensors in expanding the capabilities of imaging technology. Overall, it emphasizes the importance of integrating imaging technology with other disciplines to translate laboratory-developed technologies into applications for the natural environment.
Contribute Materials
Your contribution can guide someone’s learning journey. Share your
documents today.
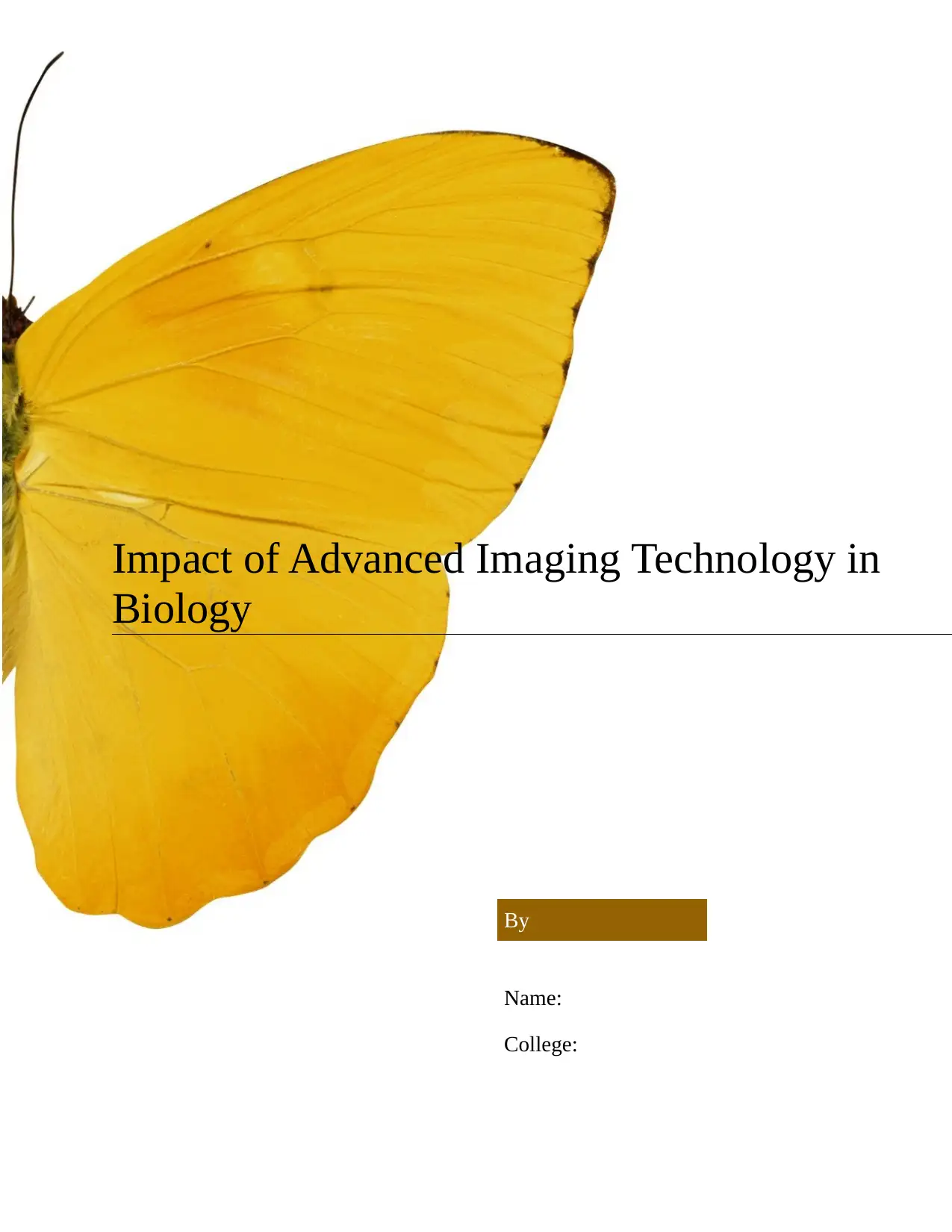
Impact of Advanced Imaging Technology in
Biology
By
Name:
College:
Biology
By
Name:
College:
Secure Best Marks with AI Grader
Need help grading? Try our AI Grader for instant feedback on your assignments.
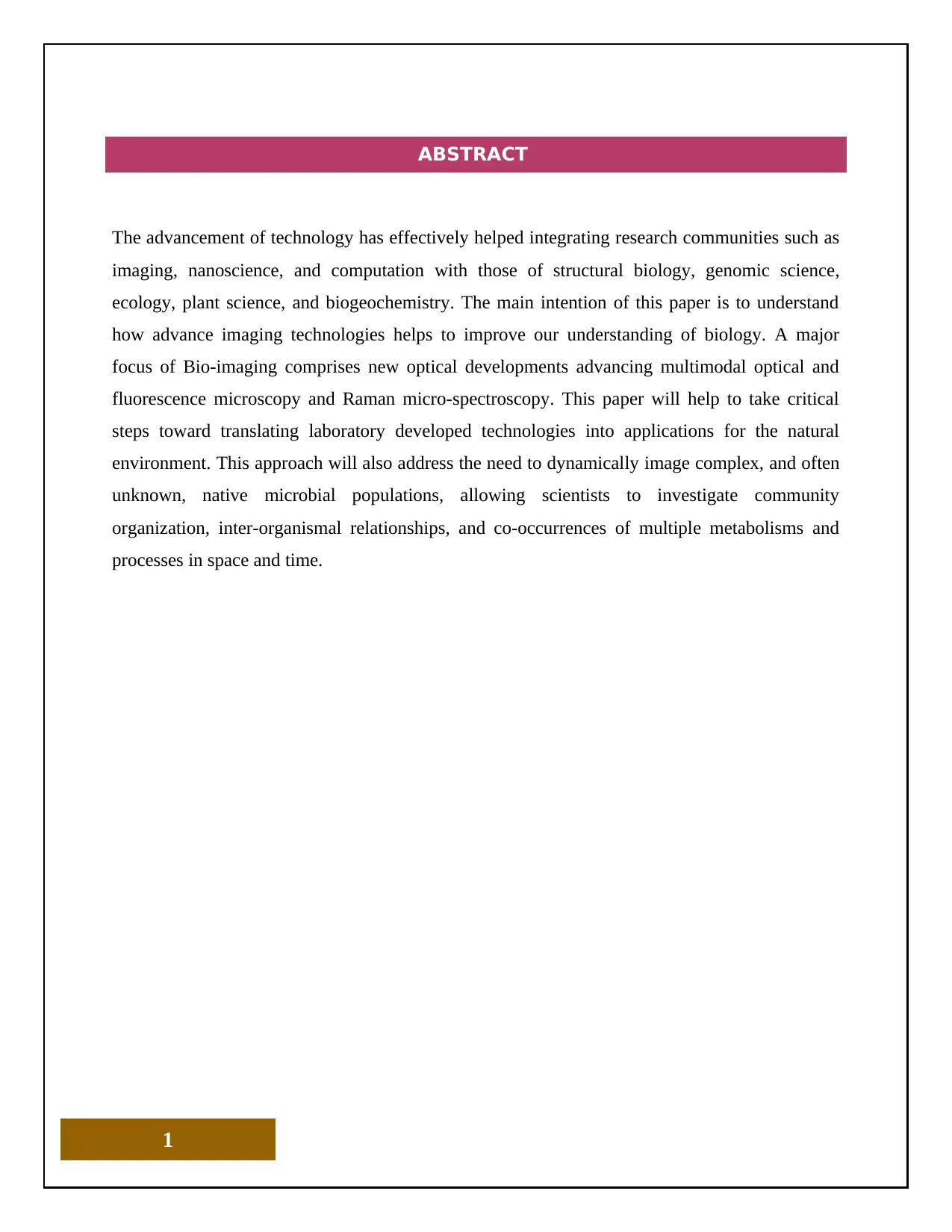
ABSTRACT
The advancement of technology has effectively helped integrating research communities such as
imaging, nanoscience, and computation with those of structural biology, genomic science,
ecology, plant science, and biogeochemistry. The main intention of this paper is to understand
how advance imaging technologies helps to improve our understanding of biology. A major
focus of Bio-imaging comprises new optical developments advancing multimodal optical and
fluorescence microscopy and Raman micro-spectroscopy. This paper will help to take critical
steps toward translating laboratory developed technologies into applications for the natural
environment. This approach will also address the need to dynamically image complex, and often
unknown, native microbial populations, allowing scientists to investigate community
organization, inter-organismal relationships, and co-occurrences of multiple metabolisms and
processes in space and time.
1
The advancement of technology has effectively helped integrating research communities such as
imaging, nanoscience, and computation with those of structural biology, genomic science,
ecology, plant science, and biogeochemistry. The main intention of this paper is to understand
how advance imaging technologies helps to improve our understanding of biology. A major
focus of Bio-imaging comprises new optical developments advancing multimodal optical and
fluorescence microscopy and Raman micro-spectroscopy. This paper will help to take critical
steps toward translating laboratory developed technologies into applications for the natural
environment. This approach will also address the need to dynamically image complex, and often
unknown, native microbial populations, allowing scientists to investigate community
organization, inter-organismal relationships, and co-occurrences of multiple metabolisms and
processes in space and time.
1
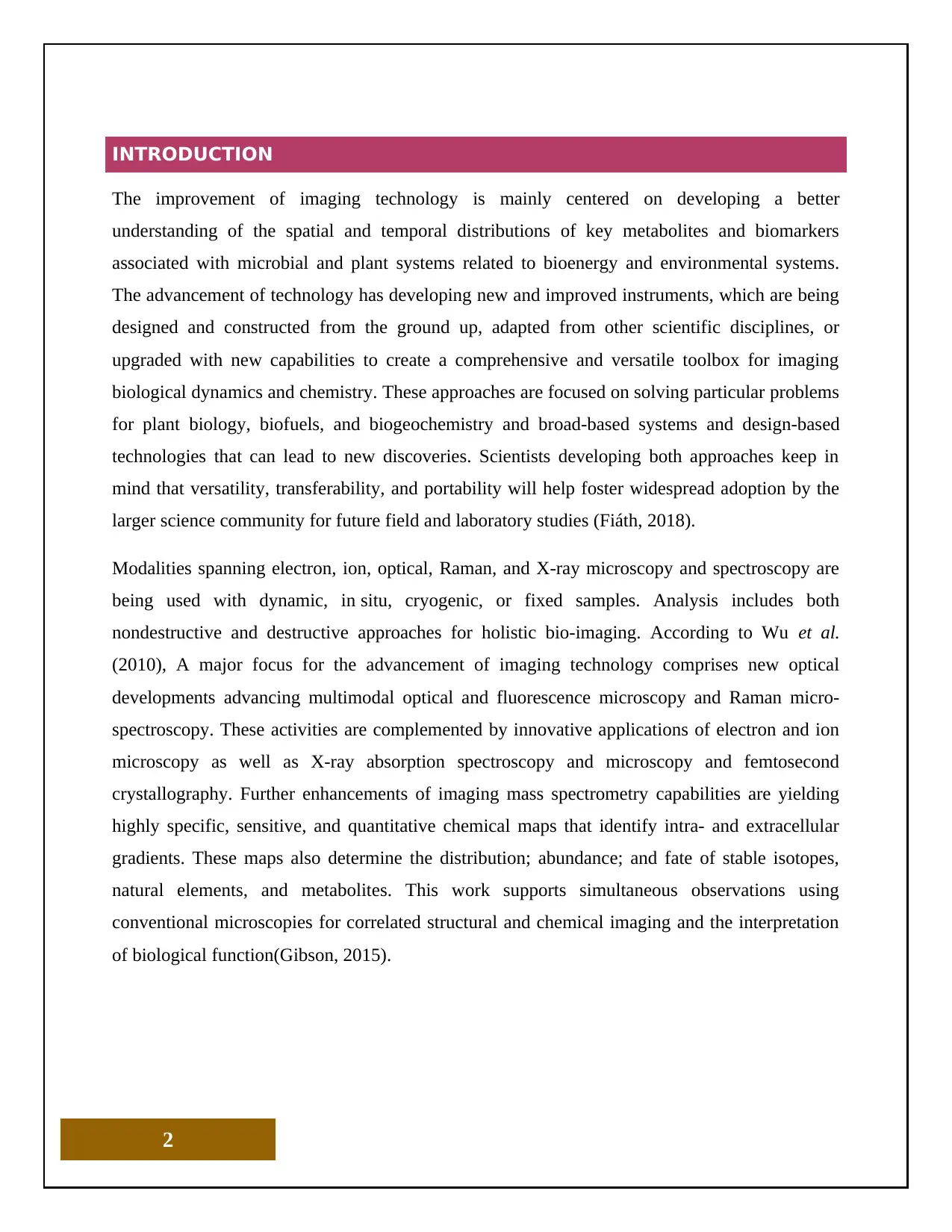
INTRODUCTION
The improvement of imaging technology is mainly centered on developing a better
understanding of the spatial and temporal distributions of key metabolites and biomarkers
associated with microbial and plant systems related to bioenergy and environmental systems.
The advancement of technology has developing new and improved instruments, which are being
designed and constructed from the ground up, adapted from other scientific disciplines, or
upgraded with new capabilities to create a comprehensive and versatile toolbox for imaging
biological dynamics and chemistry. These approaches are focused on solving particular problems
for plant biology, biofuels, and biogeochemistry and broad-based systems and design-based
technologies that can lead to new discoveries. Scientists developing both approaches keep in
mind that versatility, transferability, and portability will help foster widespread adoption by the
larger science community for future field and laboratory studies (Fiáth, 2018).
Modalities spanning electron, ion, optical, Raman, and X-ray microscopy and spectroscopy are
being used with dynamic, in situ, cryogenic, or fixed samples. Analysis includes both
nondestructive and destructive approaches for holistic bio-imaging. According to Wu et al.
(2010), A major focus for the advancement of imaging technology comprises new optical
developments advancing multimodal optical and fluorescence microscopy and Raman micro-
spectroscopy. These activities are complemented by innovative applications of electron and ion
microscopy as well as X-ray absorption spectroscopy and microscopy and femtosecond
crystallography. Further enhancements of imaging mass spectrometry capabilities are yielding
highly specific, sensitive, and quantitative chemical maps that identify intra- and extracellular
gradients. These maps also determine the distribution; abundance; and fate of stable isotopes,
natural elements, and metabolites. This work supports simultaneous observations using
conventional microscopies for correlated structural and chemical imaging and the interpretation
of biological function(Gibson, 2015).
2
The improvement of imaging technology is mainly centered on developing a better
understanding of the spatial and temporal distributions of key metabolites and biomarkers
associated with microbial and plant systems related to bioenergy and environmental systems.
The advancement of technology has developing new and improved instruments, which are being
designed and constructed from the ground up, adapted from other scientific disciplines, or
upgraded with new capabilities to create a comprehensive and versatile toolbox for imaging
biological dynamics and chemistry. These approaches are focused on solving particular problems
for plant biology, biofuels, and biogeochemistry and broad-based systems and design-based
technologies that can lead to new discoveries. Scientists developing both approaches keep in
mind that versatility, transferability, and portability will help foster widespread adoption by the
larger science community for future field and laboratory studies (Fiáth, 2018).
Modalities spanning electron, ion, optical, Raman, and X-ray microscopy and spectroscopy are
being used with dynamic, in situ, cryogenic, or fixed samples. Analysis includes both
nondestructive and destructive approaches for holistic bio-imaging. According to Wu et al.
(2010), A major focus for the advancement of imaging technology comprises new optical
developments advancing multimodal optical and fluorescence microscopy and Raman micro-
spectroscopy. These activities are complemented by innovative applications of electron and ion
microscopy as well as X-ray absorption spectroscopy and microscopy and femtosecond
crystallography. Further enhancements of imaging mass spectrometry capabilities are yielding
highly specific, sensitive, and quantitative chemical maps that identify intra- and extracellular
gradients. These maps also determine the distribution; abundance; and fate of stable isotopes,
natural elements, and metabolites. This work supports simultaneous observations using
conventional microscopies for correlated structural and chemical imaging and the interpretation
of biological function(Gibson, 2015).
2
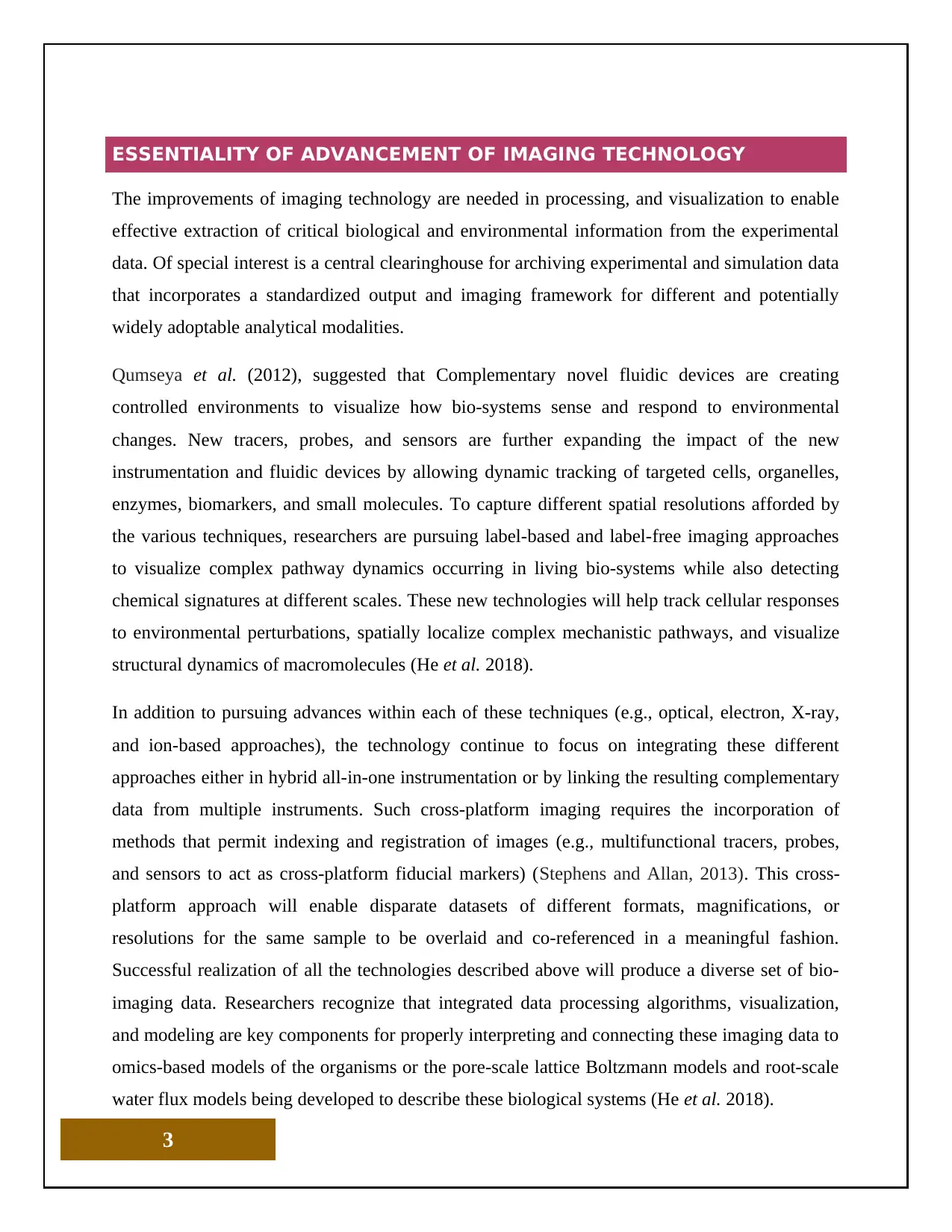
ESSENTIALITY OF ADVANCEMENT OF IMAGING TECHNOLOGY
The improvements of imaging technology are needed in processing, and visualization to enable
effective extraction of critical biological and environmental information from the experimental
data. Of special interest is a central clearinghouse for archiving experimental and simulation data
that incorporates a standardized output and imaging framework for different and potentially
widely adoptable analytical modalities.
Qumseya et al. (2012), suggested that Complementary novel fluidic devices are creating
controlled environments to visualize how bio-systems sense and respond to environmental
changes. New tracers, probes, and sensors are further expanding the impact of the new
instrumentation and fluidic devices by allowing dynamic tracking of targeted cells, organelles,
enzymes, biomarkers, and small molecules. To capture different spatial resolutions afforded by
the various techniques, researchers are pursuing label-based and label-free imaging approaches
to visualize complex pathway dynamics occurring in living bio-systems while also detecting
chemical signatures at different scales. These new technologies will help track cellular responses
to environmental perturbations, spatially localize complex mechanistic pathways, and visualize
structural dynamics of macromolecules (He et al. 2018).
In addition to pursuing advances within each of these techniques (e.g., optical, electron, X-ray,
and ion-based approaches), the technology continue to focus on integrating these different
approaches either in hybrid all-in-one instrumentation or by linking the resulting complementary
data from multiple instruments. Such cross-platform imaging requires the incorporation of
methods that permit indexing and registration of images (e.g., multifunctional tracers, probes,
and sensors to act as cross-platform fiducial markers) (Stephens and Allan, 2013). This cross-
platform approach will enable disparate datasets of different formats, magnifications, or
resolutions for the same sample to be overlaid and co-referenced in a meaningful fashion.
Successful realization of all the technologies described above will produce a diverse set of bio-
imaging data. Researchers recognize that integrated data processing algorithms, visualization,
and modeling are key components for properly interpreting and connecting these imaging data to
omics-based models of the organisms or the pore-scale lattice Boltzmann models and root-scale
water flux models being developed to describe these biological systems (He et al. 2018).
3
The improvements of imaging technology are needed in processing, and visualization to enable
effective extraction of critical biological and environmental information from the experimental
data. Of special interest is a central clearinghouse for archiving experimental and simulation data
that incorporates a standardized output and imaging framework for different and potentially
widely adoptable analytical modalities.
Qumseya et al. (2012), suggested that Complementary novel fluidic devices are creating
controlled environments to visualize how bio-systems sense and respond to environmental
changes. New tracers, probes, and sensors are further expanding the impact of the new
instrumentation and fluidic devices by allowing dynamic tracking of targeted cells, organelles,
enzymes, biomarkers, and small molecules. To capture different spatial resolutions afforded by
the various techniques, researchers are pursuing label-based and label-free imaging approaches
to visualize complex pathway dynamics occurring in living bio-systems while also detecting
chemical signatures at different scales. These new technologies will help track cellular responses
to environmental perturbations, spatially localize complex mechanistic pathways, and visualize
structural dynamics of macromolecules (He et al. 2018).
In addition to pursuing advances within each of these techniques (e.g., optical, electron, X-ray,
and ion-based approaches), the technology continue to focus on integrating these different
approaches either in hybrid all-in-one instrumentation or by linking the resulting complementary
data from multiple instruments. Such cross-platform imaging requires the incorporation of
methods that permit indexing and registration of images (e.g., multifunctional tracers, probes,
and sensors to act as cross-platform fiducial markers) (Stephens and Allan, 2013). This cross-
platform approach will enable disparate datasets of different formats, magnifications, or
resolutions for the same sample to be overlaid and co-referenced in a meaningful fashion.
Successful realization of all the technologies described above will produce a diverse set of bio-
imaging data. Researchers recognize that integrated data processing algorithms, visualization,
and modeling are key components for properly interpreting and connecting these imaging data to
omics-based models of the organisms or the pore-scale lattice Boltzmann models and root-scale
water flux models being developed to describe these biological systems (He et al. 2018).
3
Secure Best Marks with AI Grader
Need help grading? Try our AI Grader for instant feedback on your assignments.
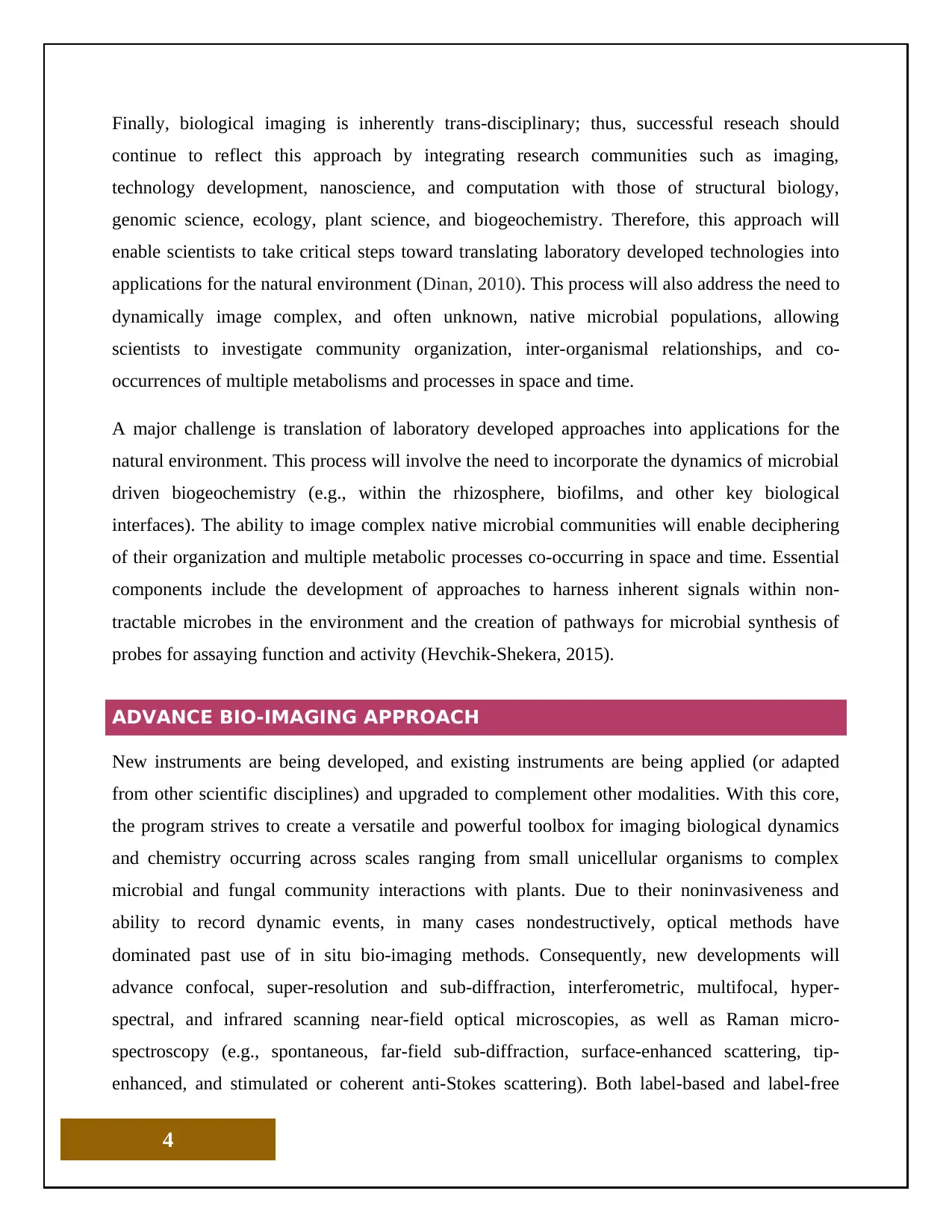
Finally, biological imaging is inherently trans-disciplinary; thus, successful reseach should
continue to reflect this approach by integrating research communities such as imaging,
technology development, nanoscience, and computation with those of structural biology,
genomic science, ecology, plant science, and biogeochemistry. Therefore, this approach will
enable scientists to take critical steps toward translating laboratory developed technologies into
applications for the natural environment (Dinan, 2010). This process will also address the need to
dynamically image complex, and often unknown, native microbial populations, allowing
scientists to investigate community organization, inter-organismal relationships, and co-
occurrences of multiple metabolisms and processes in space and time.
A major challenge is translation of laboratory developed approaches into applications for the
natural environment. This process will involve the need to incorporate the dynamics of microbial
driven biogeochemistry (e.g., within the rhizosphere, biofilms, and other key biological
interfaces). The ability to image complex native microbial communities will enable deciphering
of their organization and multiple metabolic processes co-occurring in space and time. Essential
components include the development of approaches to harness inherent signals within non-
tractable microbes in the environment and the creation of pathways for microbial synthesis of
probes for assaying function and activity (Hevchik-Shekera, 2015).
ADVANCE BIO-IMAGING APPROACH
New instruments are being developed, and existing instruments are being applied (or adapted
from other scientific disciplines) and upgraded to complement other modalities. With this core,
the program strives to create a versatile and powerful toolbox for imaging biological dynamics
and chemistry occurring across scales ranging from small unicellular organisms to complex
microbial and fungal community interactions with plants. Due to their noninvasiveness and
ability to record dynamic events, in many cases nondestructively, optical methods have
dominated past use of in situ bio-imaging methods. Consequently, new developments will
advance confocal, super-resolution and sub-diffraction, interferometric, multifocal, hyper-
spectral, and infrared scanning near-field optical microscopies, as well as Raman micro-
spectroscopy (e.g., spontaneous, far-field sub-diffraction, surface-enhanced scattering, tip-
enhanced, and stimulated or coherent anti-Stokes scattering). Both label-based and label-free
4
continue to reflect this approach by integrating research communities such as imaging,
technology development, nanoscience, and computation with those of structural biology,
genomic science, ecology, plant science, and biogeochemistry. Therefore, this approach will
enable scientists to take critical steps toward translating laboratory developed technologies into
applications for the natural environment (Dinan, 2010). This process will also address the need to
dynamically image complex, and often unknown, native microbial populations, allowing
scientists to investigate community organization, inter-organismal relationships, and co-
occurrences of multiple metabolisms and processes in space and time.
A major challenge is translation of laboratory developed approaches into applications for the
natural environment. This process will involve the need to incorporate the dynamics of microbial
driven biogeochemistry (e.g., within the rhizosphere, biofilms, and other key biological
interfaces). The ability to image complex native microbial communities will enable deciphering
of their organization and multiple metabolic processes co-occurring in space and time. Essential
components include the development of approaches to harness inherent signals within non-
tractable microbes in the environment and the creation of pathways for microbial synthesis of
probes for assaying function and activity (Hevchik-Shekera, 2015).
ADVANCE BIO-IMAGING APPROACH
New instruments are being developed, and existing instruments are being applied (or adapted
from other scientific disciplines) and upgraded to complement other modalities. With this core,
the program strives to create a versatile and powerful toolbox for imaging biological dynamics
and chemistry occurring across scales ranging from small unicellular organisms to complex
microbial and fungal community interactions with plants. Due to their noninvasiveness and
ability to record dynamic events, in many cases nondestructively, optical methods have
dominated past use of in situ bio-imaging methods. Consequently, new developments will
advance confocal, super-resolution and sub-diffraction, interferometric, multifocal, hyper-
spectral, and infrared scanning near-field optical microscopies, as well as Raman micro-
spectroscopy (e.g., spontaneous, far-field sub-diffraction, surface-enhanced scattering, tip-
enhanced, and stimulated or coherent anti-Stokes scattering). Both label-based and label-free
4
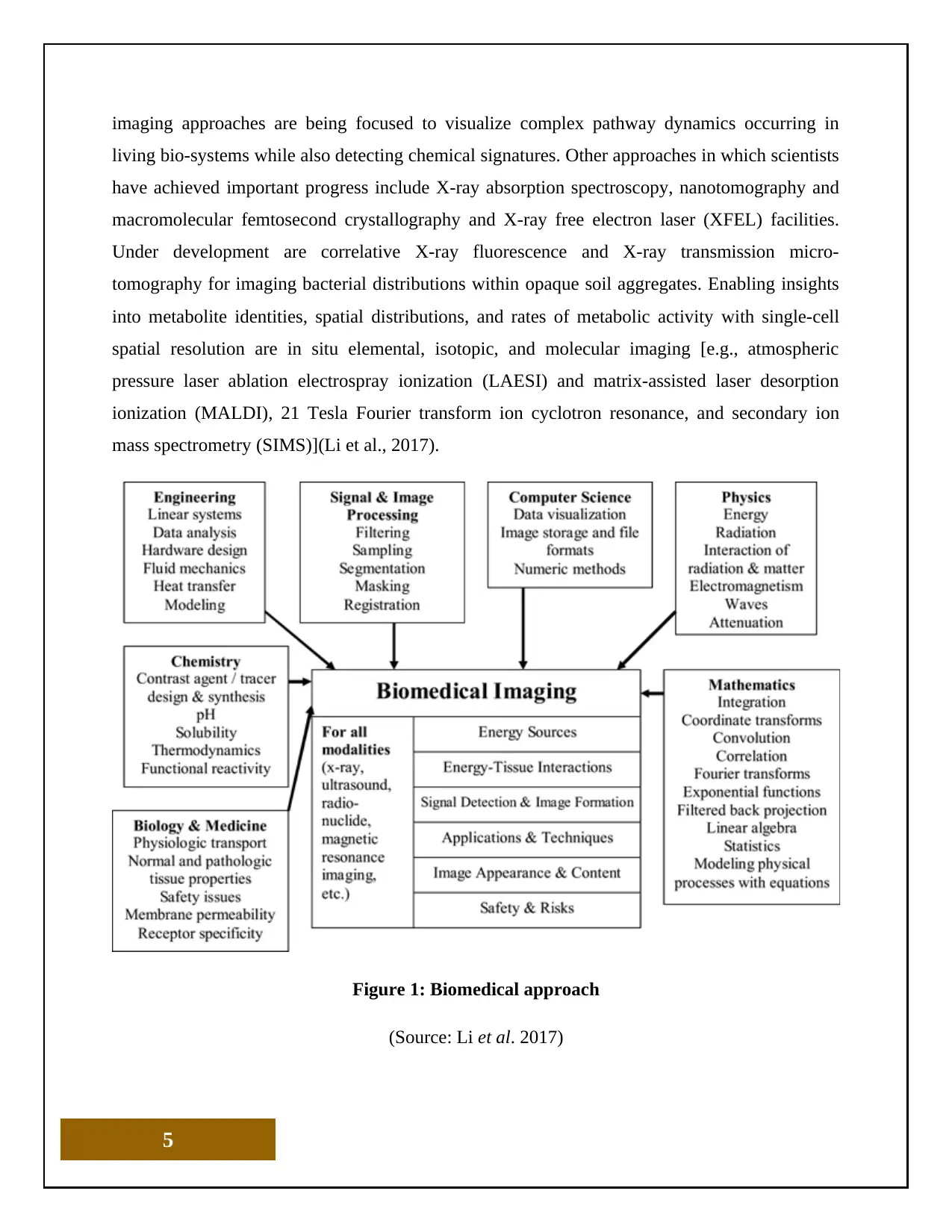
imaging approaches are being focused to visualize complex pathway dynamics occurring in
living bio-systems while also detecting chemical signatures. Other approaches in which scientists
have achieved important progress include X-ray absorption spectroscopy, nanotomography and
macromolecular femtosecond crystallography and X-ray free electron laser (XFEL) facilities.
Under development are correlative X-ray fluorescence and X-ray transmission micro-
tomography for imaging bacterial distributions within opaque soil aggregates. Enabling insights
into metabolite identities, spatial distributions, and rates of metabolic activity with single-cell
spatial resolution are in situ elemental, isotopic, and molecular imaging [e.g., atmospheric
pressure laser ablation electrospray ionization (LAESI) and matrix-assisted laser desorption
ionization (MALDI), 21 Tesla Fourier transform ion cyclotron resonance, and secondary ion
mass spectrometry (SIMS)](Li et al., 2017).
Figure 1: Biomedical approach
(Source: Li et al. 2017)
5
living bio-systems while also detecting chemical signatures. Other approaches in which scientists
have achieved important progress include X-ray absorption spectroscopy, nanotomography and
macromolecular femtosecond crystallography and X-ray free electron laser (XFEL) facilities.
Under development are correlative X-ray fluorescence and X-ray transmission micro-
tomography for imaging bacterial distributions within opaque soil aggregates. Enabling insights
into metabolite identities, spatial distributions, and rates of metabolic activity with single-cell
spatial resolution are in situ elemental, isotopic, and molecular imaging [e.g., atmospheric
pressure laser ablation electrospray ionization (LAESI) and matrix-assisted laser desorption
ionization (MALDI), 21 Tesla Fourier transform ion cyclotron resonance, and secondary ion
mass spectrometry (SIMS)](Li et al., 2017).
Figure 1: Biomedical approach
(Source: Li et al. 2017)
5
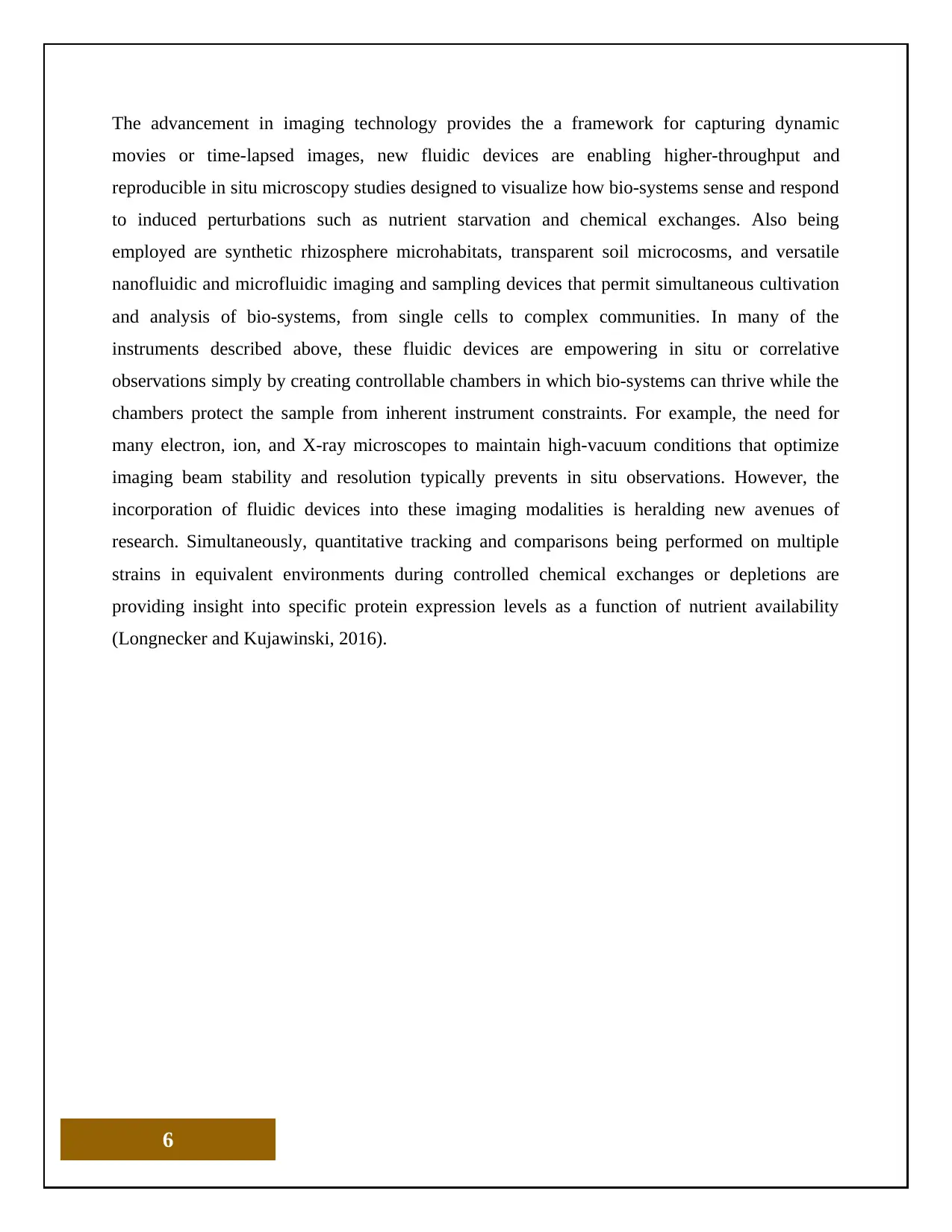
The advancement in imaging technology provides the a framework for capturing dynamic
movies or time-lapsed images, new fluidic devices are enabling higher-throughput and
reproducible in situ microscopy studies designed to visualize how bio-systems sense and respond
to induced perturbations such as nutrient starvation and chemical exchanges. Also being
employed are synthetic rhizosphere microhabitats, transparent soil microcosms, and versatile
nanofluidic and microfluidic imaging and sampling devices that permit simultaneous cultivation
and analysis of bio-systems, from single cells to complex communities. In many of the
instruments described above, these fluidic devices are empowering in situ or correlative
observations simply by creating controllable chambers in which bio-systems can thrive while the
chambers protect the sample from inherent instrument constraints. For example, the need for
many electron, ion, and X-ray microscopes to maintain high-vacuum conditions that optimize
imaging beam stability and resolution typically prevents in situ observations. However, the
incorporation of fluidic devices into these imaging modalities is heralding new avenues of
research. Simultaneously, quantitative tracking and comparisons being performed on multiple
strains in equivalent environments during controlled chemical exchanges or depletions are
providing insight into specific protein expression levels as a function of nutrient availability
(Longnecker and Kujawinski, 2016).
6
movies or time-lapsed images, new fluidic devices are enabling higher-throughput and
reproducible in situ microscopy studies designed to visualize how bio-systems sense and respond
to induced perturbations such as nutrient starvation and chemical exchanges. Also being
employed are synthetic rhizosphere microhabitats, transparent soil microcosms, and versatile
nanofluidic and microfluidic imaging and sampling devices that permit simultaneous cultivation
and analysis of bio-systems, from single cells to complex communities. In many of the
instruments described above, these fluidic devices are empowering in situ or correlative
observations simply by creating controllable chambers in which bio-systems can thrive while the
chambers protect the sample from inherent instrument constraints. For example, the need for
many electron, ion, and X-ray microscopes to maintain high-vacuum conditions that optimize
imaging beam stability and resolution typically prevents in situ observations. However, the
incorporation of fluidic devices into these imaging modalities is heralding new avenues of
research. Simultaneously, quantitative tracking and comparisons being performed on multiple
strains in equivalent environments during controlled chemical exchanges or depletions are
providing insight into specific protein expression levels as a function of nutrient availability
(Longnecker and Kujawinski, 2016).
6
Paraphrase This Document
Need a fresh take? Get an instant paraphrase of this document with our AI Paraphraser
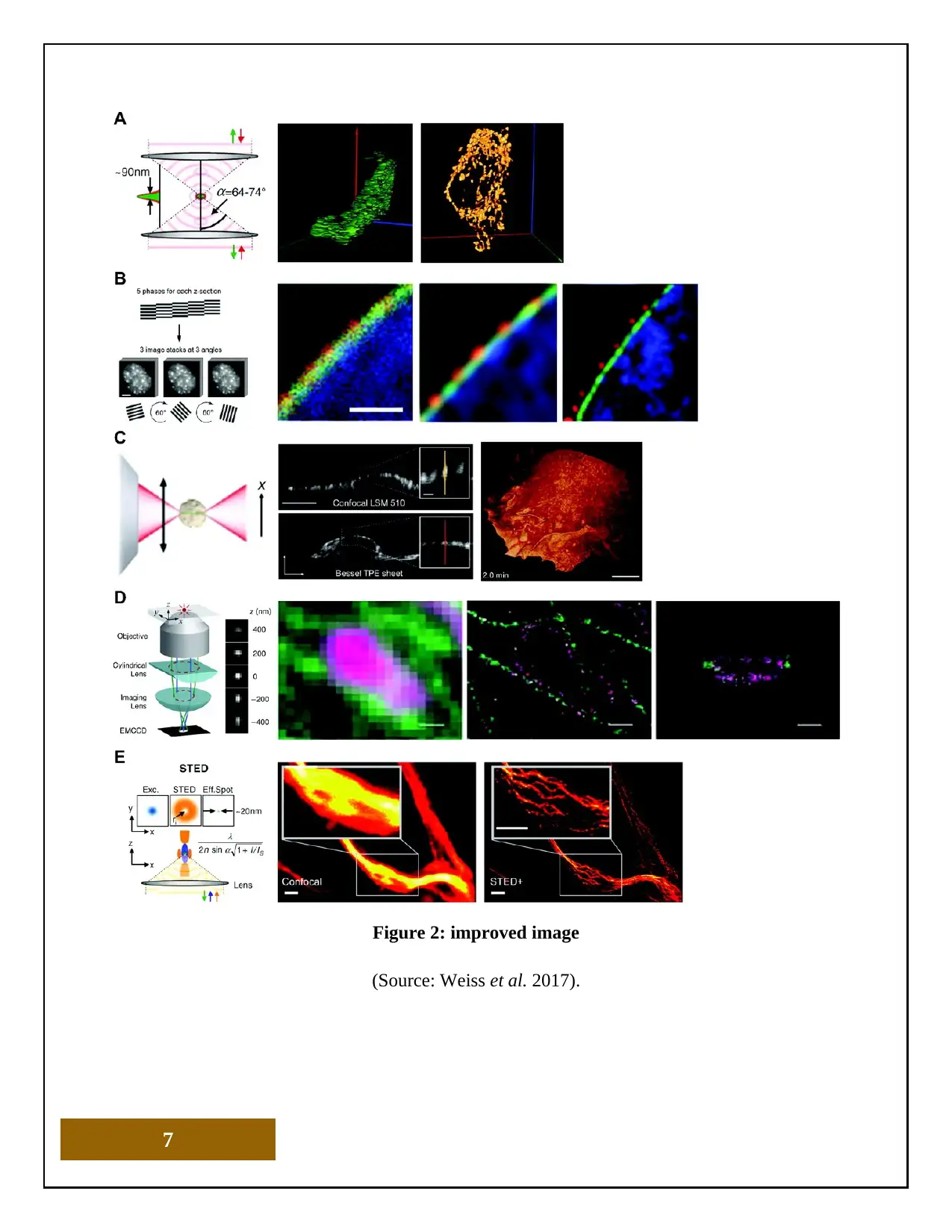
Figure 2: improved image
(Source: Weiss et al. 2017).
7
(Source: Weiss et al. 2017).
7
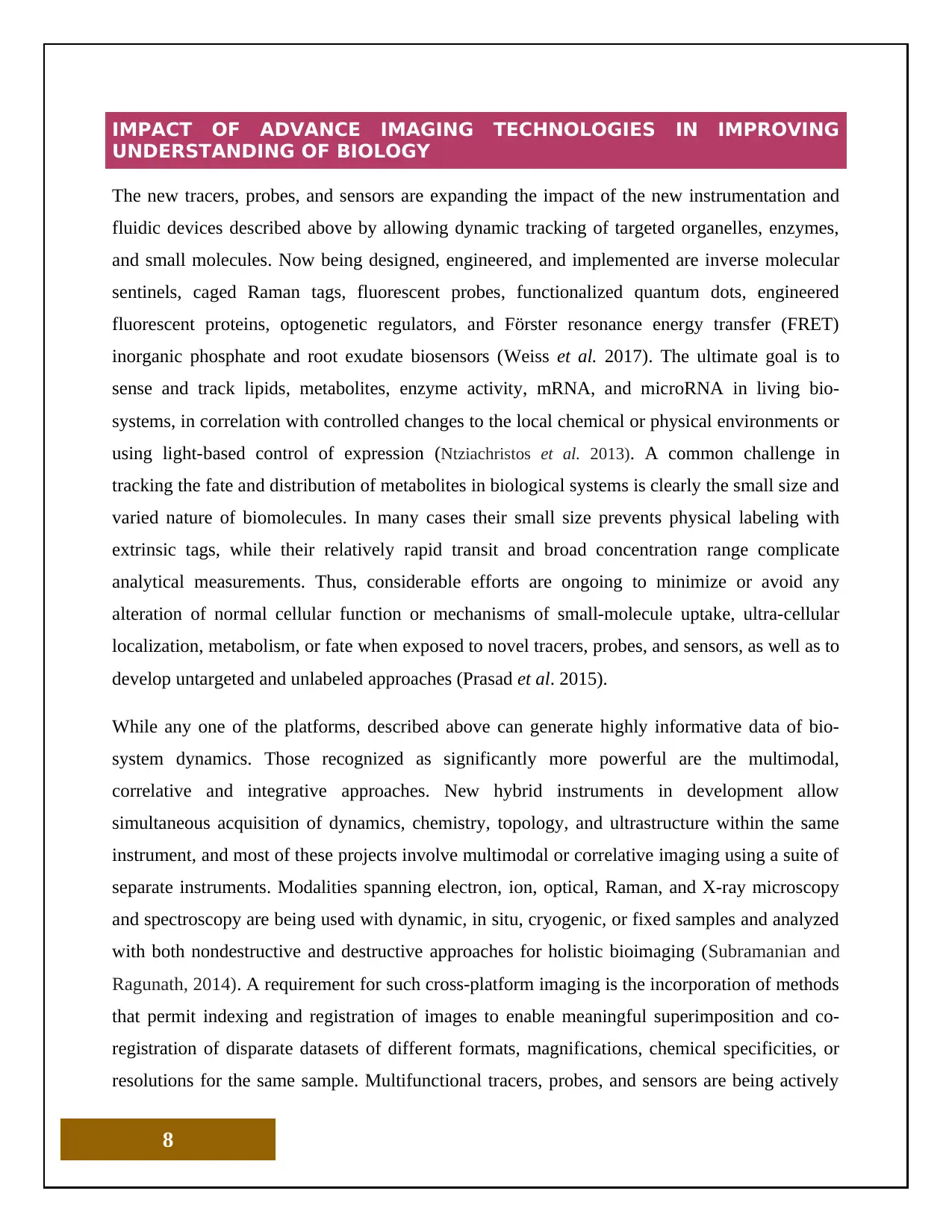
IMPACT OF ADVANCE IMAGING TECHNOLOGIES IN IMPROVING
UNDERSTANDING OF BIOLOGY
The new tracers, probes, and sensors are expanding the impact of the new instrumentation and
fluidic devices described above by allowing dynamic tracking of targeted organelles, enzymes,
and small molecules. Now being designed, engineered, and implemented are inverse molecular
sentinels, caged Raman tags, fluorescent probes, functionalized quantum dots, engineered
fluorescent proteins, optogenetic regulators, and Förster resonance energy transfer (FRET)
inorganic phosphate and root exudate biosensors (Weiss et al. 2017). The ultimate goal is to
sense and track lipids, metabolites, enzyme activity, mRNA, and microRNA in living bio-
systems, in correlation with controlled changes to the local chemical or physical environments or
using light-based control of expression (Ntziachristos et al. 2013). A common challenge in
tracking the fate and distribution of metabolites in biological systems is clearly the small size and
varied nature of biomolecules. In many cases their small size prevents physical labeling with
extrinsic tags, while their relatively rapid transit and broad concentration range complicate
analytical measurements. Thus, considerable efforts are ongoing to minimize or avoid any
alteration of normal cellular function or mechanisms of small-molecule uptake, ultra-cellular
localization, metabolism, or fate when exposed to novel tracers, probes, and sensors, as well as to
develop untargeted and unlabeled approaches (Prasad et al. 2015).
While any one of the platforms, described above can generate highly informative data of bio-
system dynamics. Those recognized as significantly more powerful are the multimodal,
correlative and integrative approaches. New hybrid instruments in development allow
simultaneous acquisition of dynamics, chemistry, topology, and ultrastructure within the same
instrument, and most of these projects involve multimodal or correlative imaging using a suite of
separate instruments. Modalities spanning electron, ion, optical, Raman, and X-ray microscopy
and spectroscopy are being used with dynamic, in situ, cryogenic, or fixed samples and analyzed
with both nondestructive and destructive approaches for holistic bioimaging (Subramanian and
Ragunath, 2014). A requirement for such cross-platform imaging is the incorporation of methods
that permit indexing and registration of images to enable meaningful superimposition and co-
registration of disparate datasets of different formats, magnifications, chemical specificities, or
resolutions for the same sample. Multifunctional tracers, probes, and sensors are being actively
8
UNDERSTANDING OF BIOLOGY
The new tracers, probes, and sensors are expanding the impact of the new instrumentation and
fluidic devices described above by allowing dynamic tracking of targeted organelles, enzymes,
and small molecules. Now being designed, engineered, and implemented are inverse molecular
sentinels, caged Raman tags, fluorescent probes, functionalized quantum dots, engineered
fluorescent proteins, optogenetic regulators, and Förster resonance energy transfer (FRET)
inorganic phosphate and root exudate biosensors (Weiss et al. 2017). The ultimate goal is to
sense and track lipids, metabolites, enzyme activity, mRNA, and microRNA in living bio-
systems, in correlation with controlled changes to the local chemical or physical environments or
using light-based control of expression (Ntziachristos et al. 2013). A common challenge in
tracking the fate and distribution of metabolites in biological systems is clearly the small size and
varied nature of biomolecules. In many cases their small size prevents physical labeling with
extrinsic tags, while their relatively rapid transit and broad concentration range complicate
analytical measurements. Thus, considerable efforts are ongoing to minimize or avoid any
alteration of normal cellular function or mechanisms of small-molecule uptake, ultra-cellular
localization, metabolism, or fate when exposed to novel tracers, probes, and sensors, as well as to
develop untargeted and unlabeled approaches (Prasad et al. 2015).
While any one of the platforms, described above can generate highly informative data of bio-
system dynamics. Those recognized as significantly more powerful are the multimodal,
correlative and integrative approaches. New hybrid instruments in development allow
simultaneous acquisition of dynamics, chemistry, topology, and ultrastructure within the same
instrument, and most of these projects involve multimodal or correlative imaging using a suite of
separate instruments. Modalities spanning electron, ion, optical, Raman, and X-ray microscopy
and spectroscopy are being used with dynamic, in situ, cryogenic, or fixed samples and analyzed
with both nondestructive and destructive approaches for holistic bioimaging (Subramanian and
Ragunath, 2014). A requirement for such cross-platform imaging is the incorporation of methods
that permit indexing and registration of images to enable meaningful superimposition and co-
registration of disparate datasets of different formats, magnifications, chemical specificities, or
resolutions for the same sample. Multifunctional tracers, probes, and sensors are being actively
8
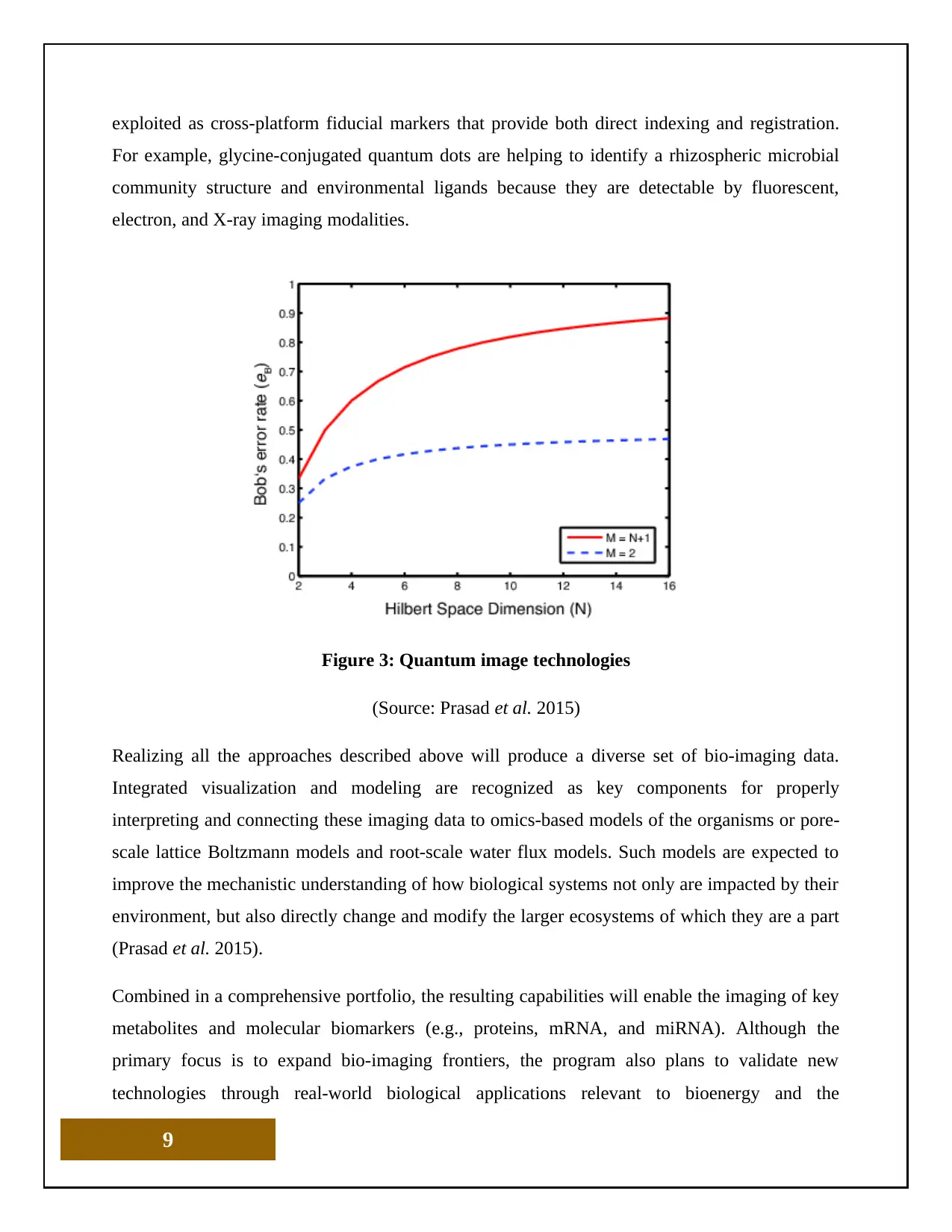
exploited as cross-platform fiducial markers that provide both direct indexing and registration.
For example, glycine-conjugated quantum dots are helping to identify a rhizospheric microbial
community structure and environmental ligands because they are detectable by fluorescent,
electron, and X-ray imaging modalities.
Figure 3: Quantum image technologies
(Source: Prasad et al. 2015)
Realizing all the approaches described above will produce a diverse set of bio-imaging data.
Integrated visualization and modeling are recognized as key components for properly
interpreting and connecting these imaging data to omics-based models of the organisms or pore-
scale lattice Boltzmann models and root-scale water flux models. Such models are expected to
improve the mechanistic understanding of how biological systems not only are impacted by their
environment, but also directly change and modify the larger ecosystems of which they are a part
(Prasad et al. 2015).
Combined in a comprehensive portfolio, the resulting capabilities will enable the imaging of key
metabolites and molecular biomarkers (e.g., proteins, mRNA, and miRNA). Although the
primary focus is to expand bio-imaging frontiers, the program also plans to validate new
technologies through real-world biological applications relevant to bioenergy and the
9
For example, glycine-conjugated quantum dots are helping to identify a rhizospheric microbial
community structure and environmental ligands because they are detectable by fluorescent,
electron, and X-ray imaging modalities.
Figure 3: Quantum image technologies
(Source: Prasad et al. 2015)
Realizing all the approaches described above will produce a diverse set of bio-imaging data.
Integrated visualization and modeling are recognized as key components for properly
interpreting and connecting these imaging data to omics-based models of the organisms or pore-
scale lattice Boltzmann models and root-scale water flux models. Such models are expected to
improve the mechanistic understanding of how biological systems not only are impacted by their
environment, but also directly change and modify the larger ecosystems of which they are a part
(Prasad et al. 2015).
Combined in a comprehensive portfolio, the resulting capabilities will enable the imaging of key
metabolites and molecular biomarkers (e.g., proteins, mRNA, and miRNA). Although the
primary focus is to expand bio-imaging frontiers, the program also plans to validate new
technologies through real-world biological applications relevant to bioenergy and the
9
Secure Best Marks with AI Grader
Need help grading? Try our AI Grader for instant feedback on your assignments.
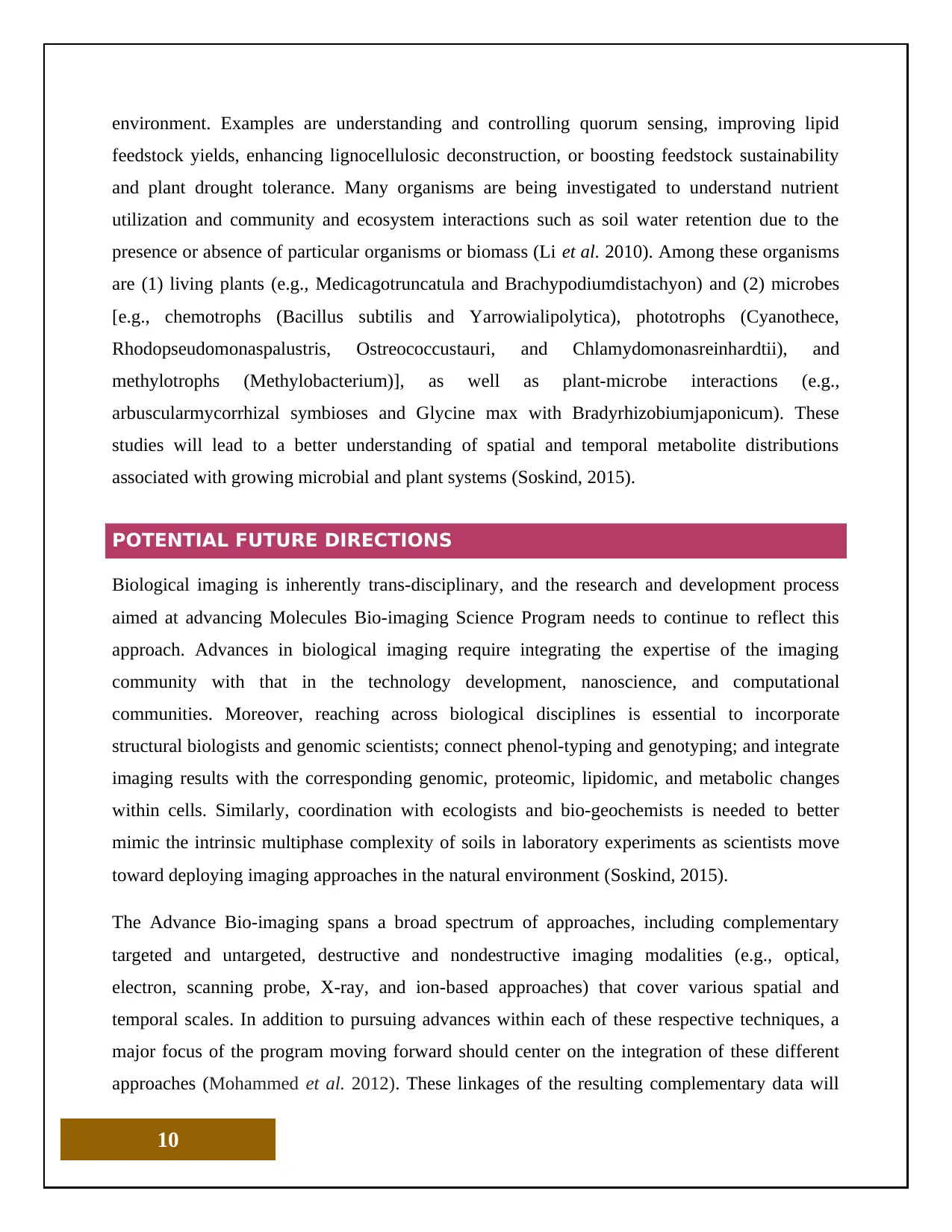
environment. Examples are understanding and controlling quorum sensing, improving lipid
feedstock yields, enhancing lignocellulosic deconstruction, or boosting feedstock sustainability
and plant drought tolerance. Many organisms are being investigated to understand nutrient
utilization and community and ecosystem interactions such as soil water retention due to the
presence or absence of particular organisms or biomass (Li et al. 2010). Among these organisms
are (1) living plants (e.g., Medicagotruncatula and Brachypodiumdistachyon) and (2) microbes
[e.g., chemotrophs (Bacillus subtilis and Yarrowialipolytica), phototrophs (Cyanothece,
Rhodopseudomonaspalustris, Ostreococcustauri, and Chlamydomonasreinhardtii), and
methylotrophs (Methylobacterium)], as well as plant-microbe interactions (e.g.,
arbuscularmycorrhizal symbioses and Glycine max with Bradyrhizobiumjaponicum). These
studies will lead to a better understanding of spatial and temporal metabolite distributions
associated with growing microbial and plant systems (Soskind, 2015).
POTENTIAL FUTURE DIRECTIONS
Biological imaging is inherently trans-disciplinary, and the research and development process
aimed at advancing Molecules Bio-imaging Science Program needs to continue to reflect this
approach. Advances in biological imaging require integrating the expertise of the imaging
community with that in the technology development, nanoscience, and computational
communities. Moreover, reaching across biological disciplines is essential to incorporate
structural biologists and genomic scientists; connect phenol-typing and genotyping; and integrate
imaging results with the corresponding genomic, proteomic, lipidomic, and metabolic changes
within cells. Similarly, coordination with ecologists and bio-geochemists is needed to better
mimic the intrinsic multiphase complexity of soils in laboratory experiments as scientists move
toward deploying imaging approaches in the natural environment (Soskind, 2015).
The Advance Bio-imaging spans a broad spectrum of approaches, including complementary
targeted and untargeted, destructive and nondestructive imaging modalities (e.g., optical,
electron, scanning probe, X-ray, and ion-based approaches) that cover various spatial and
temporal scales. In addition to pursuing advances within each of these respective techniques, a
major focus of the program moving forward should center on the integration of these different
approaches (Mohammed et al. 2012). These linkages of the resulting complementary data will
10
feedstock yields, enhancing lignocellulosic deconstruction, or boosting feedstock sustainability
and plant drought tolerance. Many organisms are being investigated to understand nutrient
utilization and community and ecosystem interactions such as soil water retention due to the
presence or absence of particular organisms or biomass (Li et al. 2010). Among these organisms
are (1) living plants (e.g., Medicagotruncatula and Brachypodiumdistachyon) and (2) microbes
[e.g., chemotrophs (Bacillus subtilis and Yarrowialipolytica), phototrophs (Cyanothece,
Rhodopseudomonaspalustris, Ostreococcustauri, and Chlamydomonasreinhardtii), and
methylotrophs (Methylobacterium)], as well as plant-microbe interactions (e.g.,
arbuscularmycorrhizal symbioses and Glycine max with Bradyrhizobiumjaponicum). These
studies will lead to a better understanding of spatial and temporal metabolite distributions
associated with growing microbial and plant systems (Soskind, 2015).
POTENTIAL FUTURE DIRECTIONS
Biological imaging is inherently trans-disciplinary, and the research and development process
aimed at advancing Molecules Bio-imaging Science Program needs to continue to reflect this
approach. Advances in biological imaging require integrating the expertise of the imaging
community with that in the technology development, nanoscience, and computational
communities. Moreover, reaching across biological disciplines is essential to incorporate
structural biologists and genomic scientists; connect phenol-typing and genotyping; and integrate
imaging results with the corresponding genomic, proteomic, lipidomic, and metabolic changes
within cells. Similarly, coordination with ecologists and bio-geochemists is needed to better
mimic the intrinsic multiphase complexity of soils in laboratory experiments as scientists move
toward deploying imaging approaches in the natural environment (Soskind, 2015).
The Advance Bio-imaging spans a broad spectrum of approaches, including complementary
targeted and untargeted, destructive and nondestructive imaging modalities (e.g., optical,
electron, scanning probe, X-ray, and ion-based approaches) that cover various spatial and
temporal scales. In addition to pursuing advances within each of these respective techniques, a
major focus of the program moving forward should center on the integration of these different
approaches (Mohammed et al. 2012). These linkages of the resulting complementary data will
10
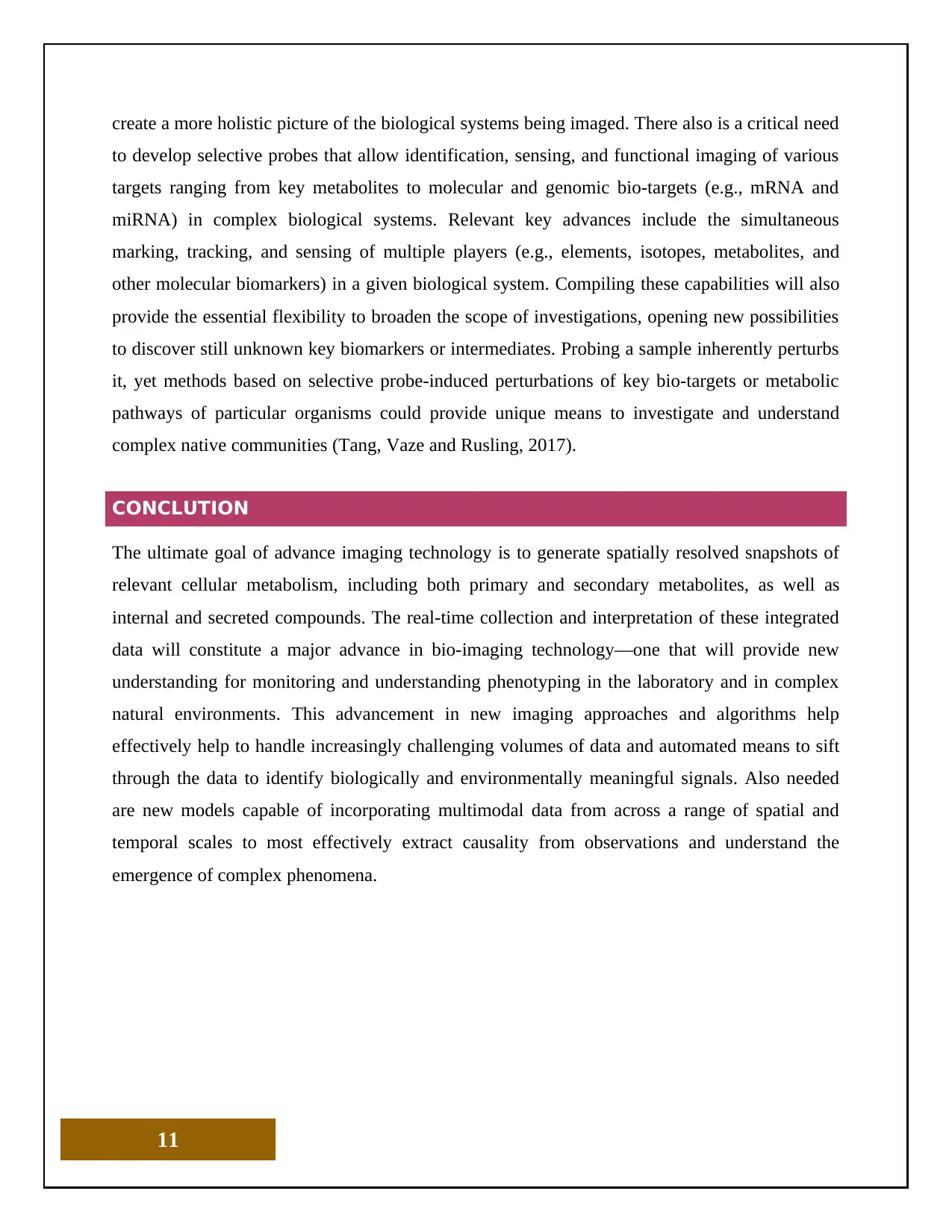
create a more holistic picture of the biological systems being imaged. There also is a critical need
to develop selective probes that allow identification, sensing, and functional imaging of various
targets ranging from key metabolites to molecular and genomic bio-targets (e.g., mRNA and
miRNA) in complex biological systems. Relevant key advances include the simultaneous
marking, tracking, and sensing of multiple players (e.g., elements, isotopes, metabolites, and
other molecular biomarkers) in a given biological system. Compiling these capabilities will also
provide the essential flexibility to broaden the scope of investigations, opening new possibilities
to discover still unknown key biomarkers or intermediates. Probing a sample inherently perturbs
it, yet methods based on selective probe-induced perturbations of key bio-targets or metabolic
pathways of particular organisms could provide unique means to investigate and understand
complex native communities (Tang, Vaze and Rusling, 2017).
CONCLUTION
The ultimate goal of advance imaging technology is to generate spatially resolved snapshots of
relevant cellular metabolism, including both primary and secondary metabolites, as well as
internal and secreted compounds. The real-time collection and interpretation of these integrated
data will constitute a major advance in bio-imaging technology—one that will provide new
understanding for monitoring and understanding phenotyping in the laboratory and in complex
natural environments. This advancement in new imaging approaches and algorithms help
effectively help to handle increasingly challenging volumes of data and automated means to sift
through the data to identify biologically and environmentally meaningful signals. Also needed
are new models capable of incorporating multimodal data from across a range of spatial and
temporal scales to most effectively extract causality from observations and understand the
emergence of complex phenomena.
11
to develop selective probes that allow identification, sensing, and functional imaging of various
targets ranging from key metabolites to molecular and genomic bio-targets (e.g., mRNA and
miRNA) in complex biological systems. Relevant key advances include the simultaneous
marking, tracking, and sensing of multiple players (e.g., elements, isotopes, metabolites, and
other molecular biomarkers) in a given biological system. Compiling these capabilities will also
provide the essential flexibility to broaden the scope of investigations, opening new possibilities
to discover still unknown key biomarkers or intermediates. Probing a sample inherently perturbs
it, yet methods based on selective probe-induced perturbations of key bio-targets or metabolic
pathways of particular organisms could provide unique means to investigate and understand
complex native communities (Tang, Vaze and Rusling, 2017).
CONCLUTION
The ultimate goal of advance imaging technology is to generate spatially resolved snapshots of
relevant cellular metabolism, including both primary and secondary metabolites, as well as
internal and secreted compounds. The real-time collection and interpretation of these integrated
data will constitute a major advance in bio-imaging technology—one that will provide new
understanding for monitoring and understanding phenotyping in the laboratory and in complex
natural environments. This advancement in new imaging approaches and algorithms help
effectively help to handle increasingly challenging volumes of data and automated means to sift
through the data to identify biologically and environmentally meaningful signals. Also needed
are new models capable of incorporating multimodal data from across a range of spatial and
temporal scales to most effectively extract causality from observations and understand the
emergence of complex phenomena.
11
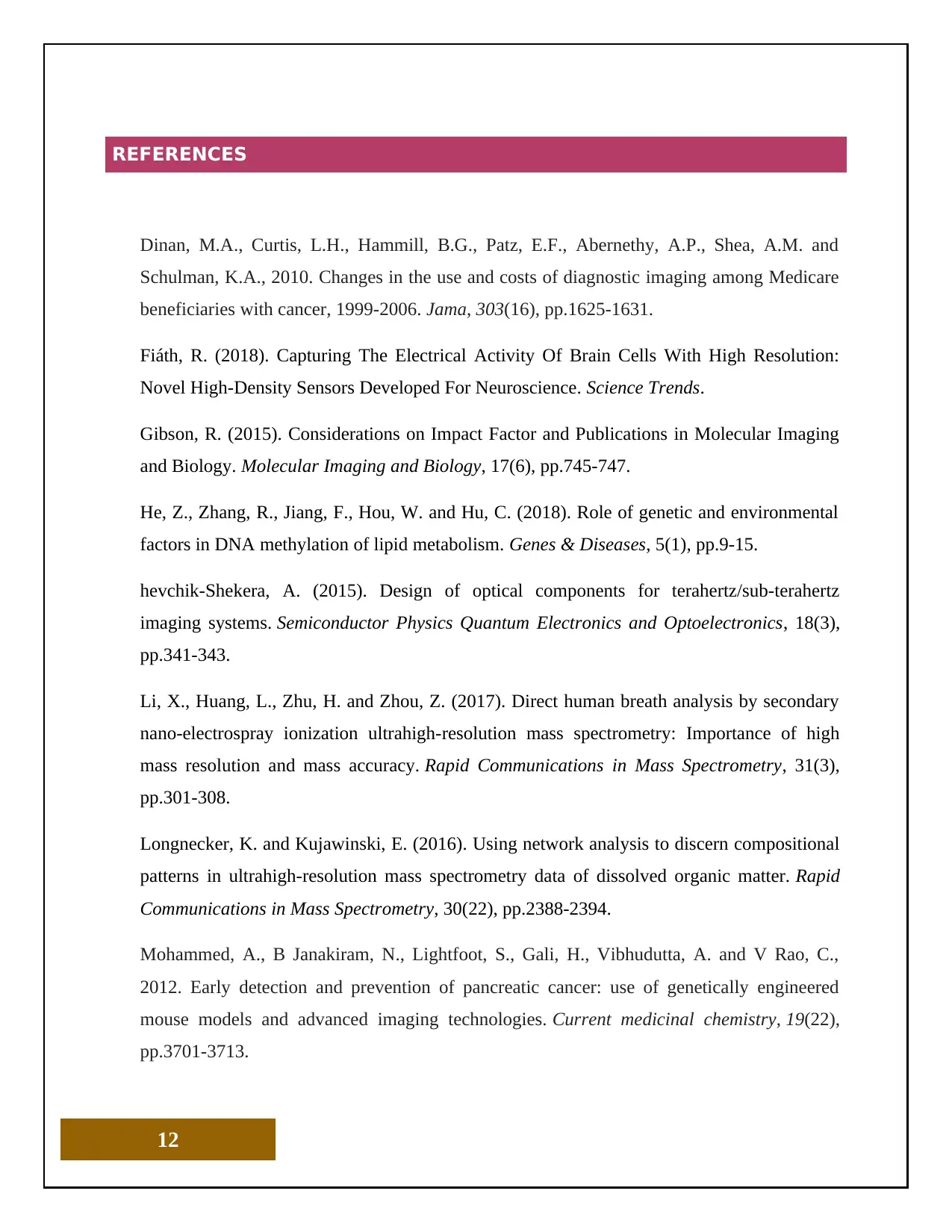
REFERENCES
Dinan, M.A., Curtis, L.H., Hammill, B.G., Patz, E.F., Abernethy, A.P., Shea, A.M. and
Schulman, K.A., 2010. Changes in the use and costs of diagnostic imaging among Medicare
beneficiaries with cancer, 1999-2006. Jama, 303(16), pp.1625-1631.
Fiáth, R. (2018). Capturing The Electrical Activity Of Brain Cells With High Resolution:
Novel High-Density Sensors Developed For Neuroscience. Science Trends.
Gibson, R. (2015). Considerations on Impact Factor and Publications in Molecular Imaging
and Biology. Molecular Imaging and Biology, 17(6), pp.745-747.
He, Z., Zhang, R., Jiang, F., Hou, W. and Hu, C. (2018). Role of genetic and environmental
factors in DNA methylation of lipid metabolism. Genes & Diseases, 5(1), pp.9-15.
hevchik-Shekera, A. (2015). Design of optical components for terahertz/sub-terahertz
imaging systems. Semiconductor Physics Quantum Electronics and Optoelectronics, 18(3),
pp.341-343.
Li, X., Huang, L., Zhu, H. and Zhou, Z. (2017). Direct human breath analysis by secondary
nano-electrospray ionization ultrahigh-resolution mass spectrometry: Importance of high
mass resolution and mass accuracy. Rapid Communications in Mass Spectrometry, 31(3),
pp.301-308.
Longnecker, K. and Kujawinski, E. (2016). Using network analysis to discern compositional
patterns in ultrahigh-resolution mass spectrometry data of dissolved organic matter. Rapid
Communications in Mass Spectrometry, 30(22), pp.2388-2394.
Mohammed, A., B Janakiram, N., Lightfoot, S., Gali, H., Vibhudutta, A. and V Rao, C.,
2012. Early detection and prevention of pancreatic cancer: use of genetically engineered
mouse models and advanced imaging technologies. Current medicinal chemistry, 19(22),
pp.3701-3713.
12
Dinan, M.A., Curtis, L.H., Hammill, B.G., Patz, E.F., Abernethy, A.P., Shea, A.M. and
Schulman, K.A., 2010. Changes in the use and costs of diagnostic imaging among Medicare
beneficiaries with cancer, 1999-2006. Jama, 303(16), pp.1625-1631.
Fiáth, R. (2018). Capturing The Electrical Activity Of Brain Cells With High Resolution:
Novel High-Density Sensors Developed For Neuroscience. Science Trends.
Gibson, R. (2015). Considerations on Impact Factor and Publications in Molecular Imaging
and Biology. Molecular Imaging and Biology, 17(6), pp.745-747.
He, Z., Zhang, R., Jiang, F., Hou, W. and Hu, C. (2018). Role of genetic and environmental
factors in DNA methylation of lipid metabolism. Genes & Diseases, 5(1), pp.9-15.
hevchik-Shekera, A. (2015). Design of optical components for terahertz/sub-terahertz
imaging systems. Semiconductor Physics Quantum Electronics and Optoelectronics, 18(3),
pp.341-343.
Li, X., Huang, L., Zhu, H. and Zhou, Z. (2017). Direct human breath analysis by secondary
nano-electrospray ionization ultrahigh-resolution mass spectrometry: Importance of high
mass resolution and mass accuracy. Rapid Communications in Mass Spectrometry, 31(3),
pp.301-308.
Longnecker, K. and Kujawinski, E. (2016). Using network analysis to discern compositional
patterns in ultrahigh-resolution mass spectrometry data of dissolved organic matter. Rapid
Communications in Mass Spectrometry, 30(22), pp.2388-2394.
Mohammed, A., B Janakiram, N., Lightfoot, S., Gali, H., Vibhudutta, A. and V Rao, C.,
2012. Early detection and prevention of pancreatic cancer: use of genetically engineered
mouse models and advanced imaging technologies. Current medicinal chemistry, 19(22),
pp.3701-3713.
12
Paraphrase This Document
Need a fresh take? Get an instant paraphrase of this document with our AI Paraphraser
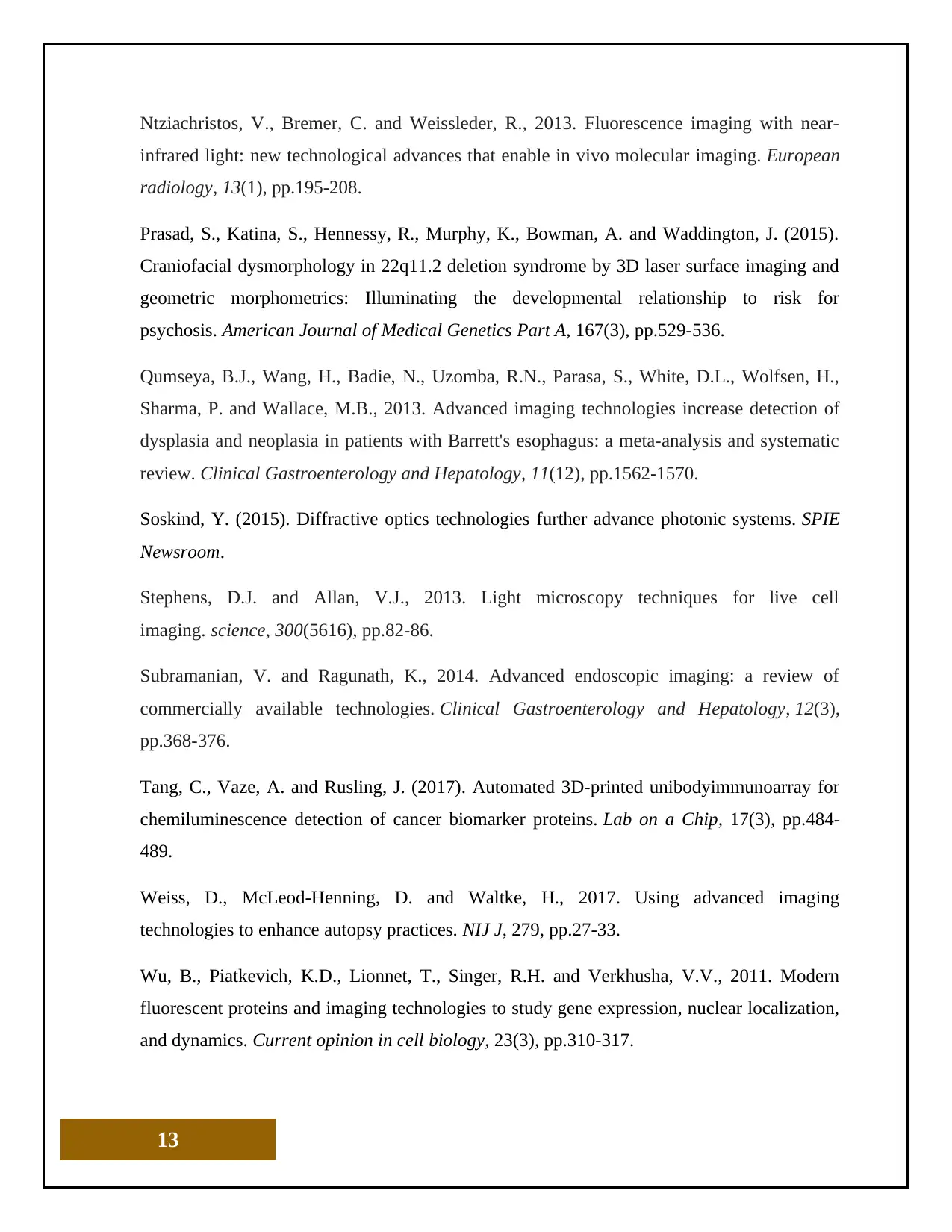
Ntziachristos, V., Bremer, C. and Weissleder, R., 2013. Fluorescence imaging with near-
infrared light: new technological advances that enable in vivo molecular imaging. European
radiology, 13(1), pp.195-208.
Prasad, S., Katina, S., Hennessy, R., Murphy, K., Bowman, A. and Waddington, J. (2015).
Craniofacial dysmorphology in 22q11.2 deletion syndrome by 3D laser surface imaging and
geometric morphometrics: Illuminating the developmental relationship to risk for
psychosis. American Journal of Medical Genetics Part A, 167(3), pp.529-536.
Qumseya, B.J., Wang, H., Badie, N., Uzomba, R.N., Parasa, S., White, D.L., Wolfsen, H.,
Sharma, P. and Wallace, M.B., 2013. Advanced imaging technologies increase detection of
dysplasia and neoplasia in patients with Barrett's esophagus: a meta-analysis and systematic
review. Clinical Gastroenterology and Hepatology, 11(12), pp.1562-1570.
Soskind, Y. (2015). Diffractive optics technologies further advance photonic systems. SPIE
Newsroom.
Stephens, D.J. and Allan, V.J., 2013. Light microscopy techniques for live cell
imaging. science, 300(5616), pp.82-86.
Subramanian, V. and Ragunath, K., 2014. Advanced endoscopic imaging: a review of
commercially available technologies. Clinical Gastroenterology and Hepatology, 12(3),
pp.368-376.
Tang, C., Vaze, A. and Rusling, J. (2017). Automated 3D-printed unibodyimmunoarray for
chemiluminescence detection of cancer biomarker proteins. Lab on a Chip, 17(3), pp.484-
489.
Weiss, D., McLeod-Henning, D. and Waltke, H., 2017. Using advanced imaging
technologies to enhance autopsy practices. NIJ J, 279, pp.27-33.
Wu, B., Piatkevich, K.D., Lionnet, T., Singer, R.H. and Verkhusha, V.V., 2011. Modern
fluorescent proteins and imaging technologies to study gene expression, nuclear localization,
and dynamics. Current opinion in cell biology, 23(3), pp.310-317.
13
infrared light: new technological advances that enable in vivo molecular imaging. European
radiology, 13(1), pp.195-208.
Prasad, S., Katina, S., Hennessy, R., Murphy, K., Bowman, A. and Waddington, J. (2015).
Craniofacial dysmorphology in 22q11.2 deletion syndrome by 3D laser surface imaging and
geometric morphometrics: Illuminating the developmental relationship to risk for
psychosis. American Journal of Medical Genetics Part A, 167(3), pp.529-536.
Qumseya, B.J., Wang, H., Badie, N., Uzomba, R.N., Parasa, S., White, D.L., Wolfsen, H.,
Sharma, P. and Wallace, M.B., 2013. Advanced imaging technologies increase detection of
dysplasia and neoplasia in patients with Barrett's esophagus: a meta-analysis and systematic
review. Clinical Gastroenterology and Hepatology, 11(12), pp.1562-1570.
Soskind, Y. (2015). Diffractive optics technologies further advance photonic systems. SPIE
Newsroom.
Stephens, D.J. and Allan, V.J., 2013. Light microscopy techniques for live cell
imaging. science, 300(5616), pp.82-86.
Subramanian, V. and Ragunath, K., 2014. Advanced endoscopic imaging: a review of
commercially available technologies. Clinical Gastroenterology and Hepatology, 12(3),
pp.368-376.
Tang, C., Vaze, A. and Rusling, J. (2017). Automated 3D-printed unibodyimmunoarray for
chemiluminescence detection of cancer biomarker proteins. Lab on a Chip, 17(3), pp.484-
489.
Weiss, D., McLeod-Henning, D. and Waltke, H., 2017. Using advanced imaging
technologies to enhance autopsy practices. NIJ J, 279, pp.27-33.
Wu, B., Piatkevich, K.D., Lionnet, T., Singer, R.H. and Verkhusha, V.V., 2011. Modern
fluorescent proteins and imaging technologies to study gene expression, nuclear localization,
and dynamics. Current opinion in cell biology, 23(3), pp.310-317.
13
1 out of 14
![[object Object]](/_next/image/?url=%2F_next%2Fstatic%2Fmedia%2Flogo.6d15ce61.png&w=640&q=75)
Your All-in-One AI-Powered Toolkit for Academic Success.
+13062052269
info@desklib.com
Available 24*7 on WhatsApp / Email
Unlock your academic potential
© 2024 | Zucol Services PVT LTD | All rights reserved.