Environmental Engineering Project: SBR Simulation for Nutrient Removal
VerifiedAdded on 2022/08/25
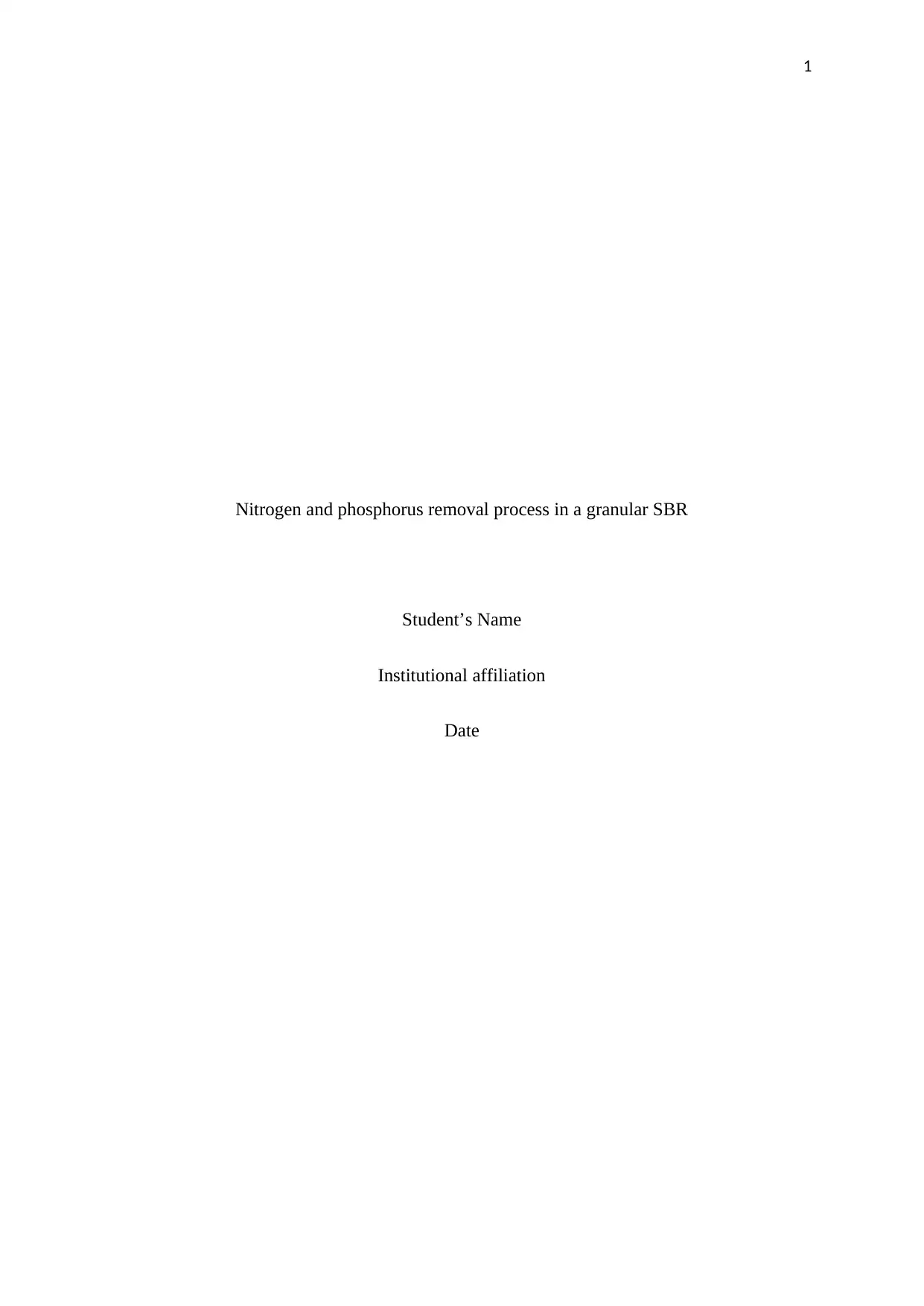
Nitrogen and phosphorus removal process in a granular SBR
Student’s Name
Institutional affiliation
Date
Paraphrase This Document
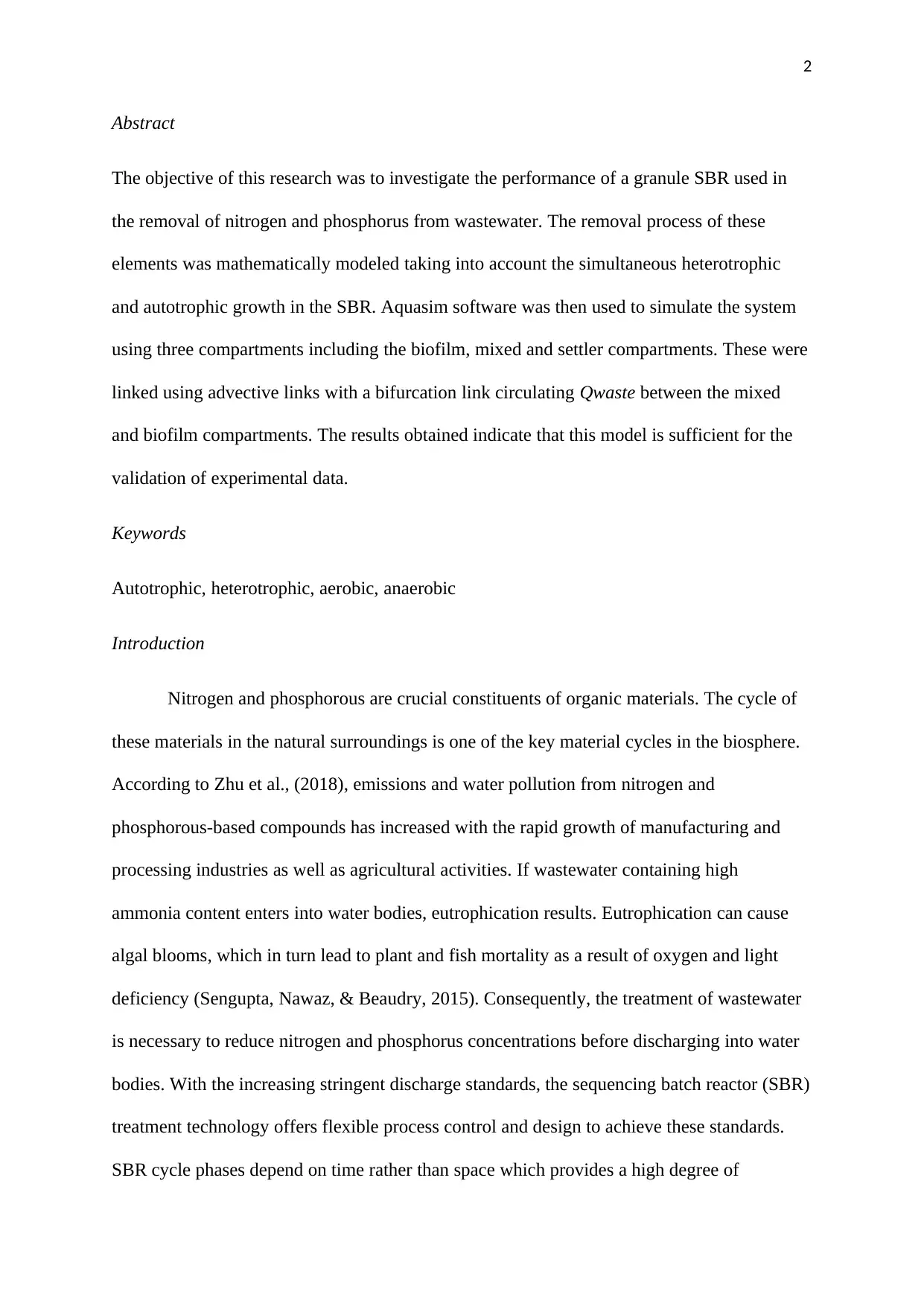
Abstract
The objective of this research was to investigate the performance of a granule SBR used in
the removal of nitrogen and phosphorus from wastewater. The removal process of these
elements was mathematically modeled taking into account the simultaneous heterotrophic
and autotrophic growth in the SBR. Aquasim software was then used to simulate the system
using three compartments including the biofilm, mixed and settler compartments. These were
linked using advective links with a bifurcation link circulating Qwaste between the mixed
and biofilm compartments. The results obtained indicate that this model is sufficient for the
validation of experimental data.
Keywords
Autotrophic, heterotrophic, aerobic, anaerobic
Introduction
Nitrogen and phosphorous are crucial constituents of organic materials. The cycle of
these materials in the natural surroundings is one of the key material cycles in the biosphere.
According to Zhu et al., (2018), emissions and water pollution from nitrogen and
phosphorous-based compounds has increased with the rapid growth of manufacturing and
processing industries as well as agricultural activities. If wastewater containing high
ammonia content enters into water bodies, eutrophication results. Eutrophication can cause
algal blooms, which in turn lead to plant and fish mortality as a result of oxygen and light
deficiency (Sengupta, Nawaz, & Beaudry, 2015). Consequently, the treatment of wastewater
is necessary to reduce nitrogen and phosphorus concentrations before discharging into water
bodies. With the increasing stringent discharge standards, the sequencing batch reactor (SBR)
treatment technology offers flexible process control and design to achieve these standards.
SBR cycle phases depend on time rather than space which provides a high degree of
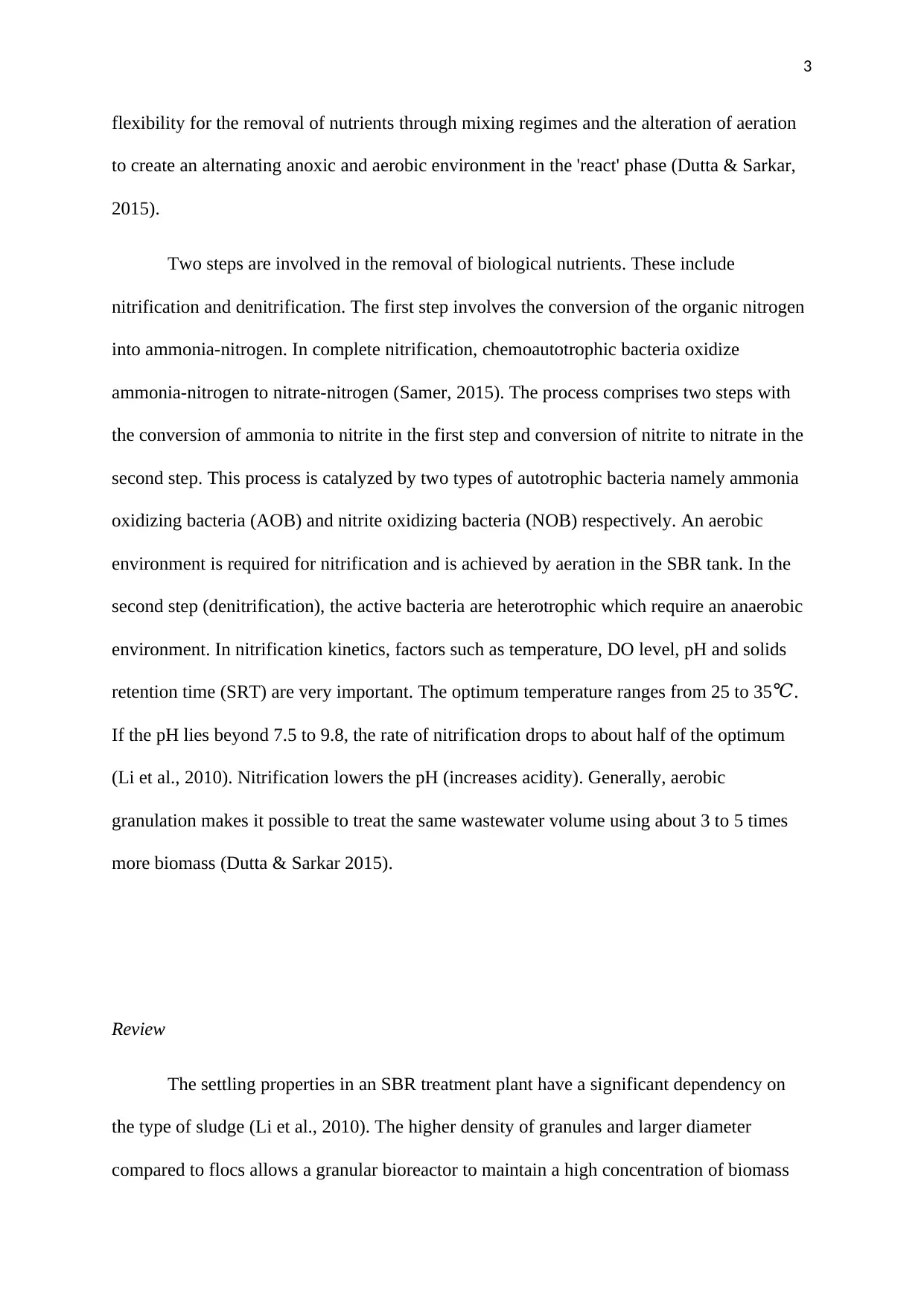
flexibility for the removal of nutrients through mixing regimes and the alteration of aeration
to create an alternating anoxic and aerobic environment in the 'react' phase (Dutta & Sarkar,
2015).
Two steps are involved in the removal of biological nutrients. These include
nitrification and denitrification. The first step involves the conversion of the organic nitrogen
into ammonia-nitrogen. In complete nitrification, chemoautotrophic bacteria oxidize
ammonia-nitrogen to nitrate-nitrogen (Samer, 2015). The process comprises two steps with
the conversion of ammonia to nitrite in the first step and conversion of nitrite to nitrate in the
second step. This process is catalyzed by two types of autotrophic bacteria namely ammonia
oxidizing bacteria (AOB) and nitrite oxidizing bacteria (NOB) respectively. An aerobic
environment is required for nitrification and is achieved by aeration in the SBR tank. In the
second step (denitrification), the active bacteria are heterotrophic which require an anaerobic
environment. In nitrification kinetics, factors such as temperature, DO level, pH and solids
retention time (SRT) are very important. The optimum temperature ranges from 25 to 35℃.
If the pH lies beyond 7.5 to 9.8, the rate of nitrification drops to about half of the optimum
(Li et al., 2010). Nitrification lowers the pH (increases acidity). Generally, aerobic
granulation makes it possible to treat the same wastewater volume using about 3 to 5 times
more biomass (Dutta & Sarkar 2015).
Review
The settling properties in an SBR treatment plant have a significant dependency on
the type of sludge (Li et al., 2010). The higher density of granules and larger diameter
compared to flocs allows a granular bioreactor to maintain a high concentration of biomass
⊘ This is a preview!⊘
Do you want full access?
Subscribe today to unlock all pages.

Trusted by 1+ million students worldwide
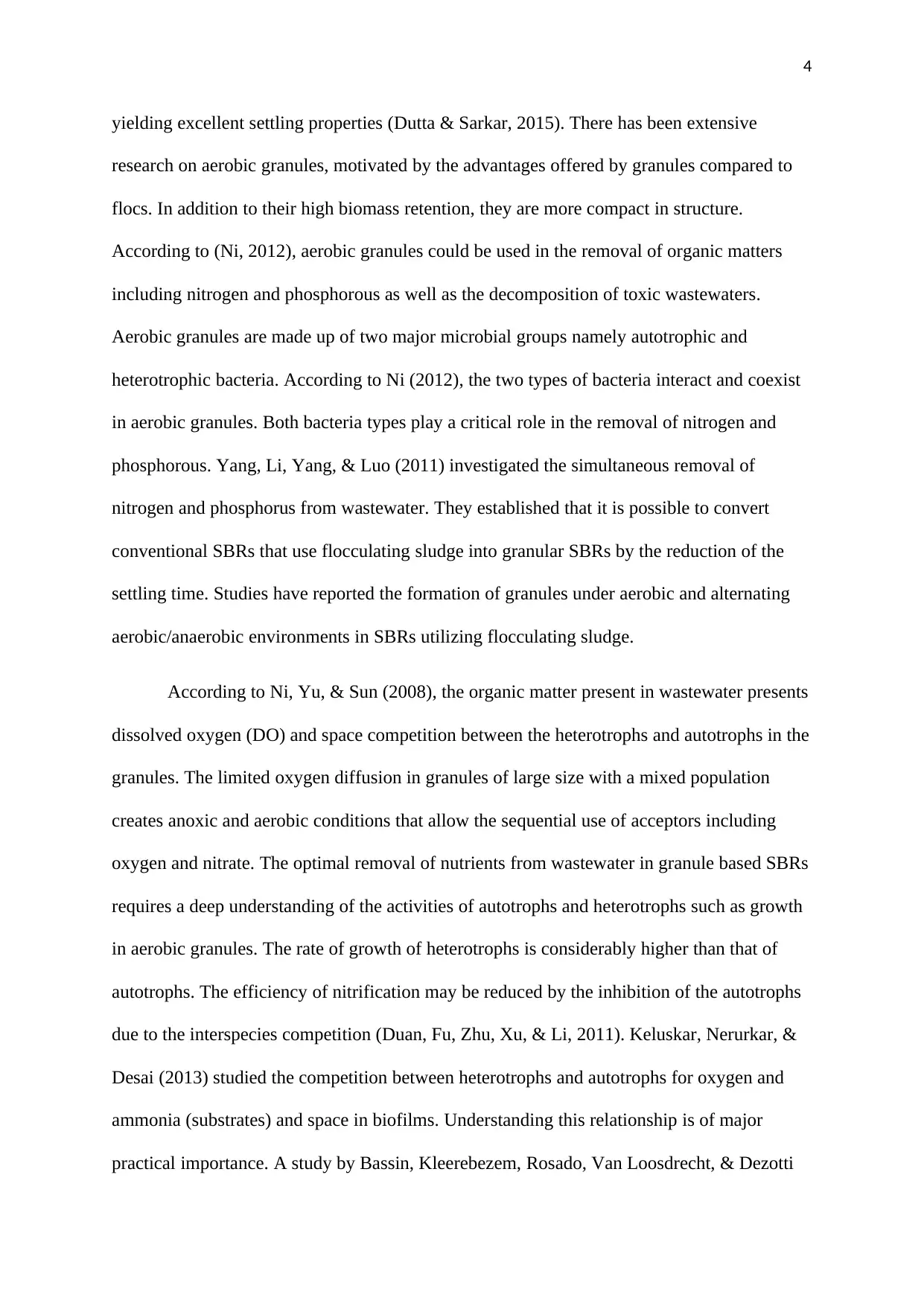
yielding excellent settling properties (Dutta & Sarkar, 2015). There has been extensive
research on aerobic granules, motivated by the advantages offered by granules compared to
flocs. In addition to their high biomass retention, they are more compact in structure.
According to (Ni, 2012), aerobic granules could be used in the removal of organic matters
including nitrogen and phosphorous as well as the decomposition of toxic wastewaters.
Aerobic granules are made up of two major microbial groups namely autotrophic and
heterotrophic bacteria. According to Ni (2012), the two types of bacteria interact and coexist
in aerobic granules. Both bacteria types play a critical role in the removal of nitrogen and
phosphorous. Yang, Li, Yang, & Luo (2011) investigated the simultaneous removal of
nitrogen and phosphorus from wastewater. They established that it is possible to convert
conventional SBRs that use flocculating sludge into granular SBRs by the reduction of the
settling time. Studies have reported the formation of granules under aerobic and alternating
aerobic/anaerobic environments in SBRs utilizing flocculating sludge.
According to Ni, Yu, & Sun (2008), the organic matter present in wastewater presents
dissolved oxygen (DO) and space competition between the heterotrophs and autotrophs in the
granules. The limited oxygen diffusion in granules of large size with a mixed population
creates anoxic and aerobic conditions that allow the sequential use of acceptors including
oxygen and nitrate. The optimal removal of nutrients from wastewater in granule based SBRs
requires a deep understanding of the activities of autotrophs and heterotrophs such as growth
in aerobic granules. The rate of growth of heterotrophs is considerably higher than that of
autotrophs. The efficiency of nitrification may be reduced by the inhibition of the autotrophs
due to the interspecies competition (Duan, Fu, Zhu, Xu, & Li, 2011). Keluskar, Nerurkar, &
Desai (2013) studied the competition between heterotrophs and autotrophs for oxygen and
ammonia (substrates) and space in biofilms. Understanding this relationship is of major
practical importance. A study by Bassin, Kleerebezem, Rosado, Van Loosdrecht, & Dezotti
Paraphrase This Document
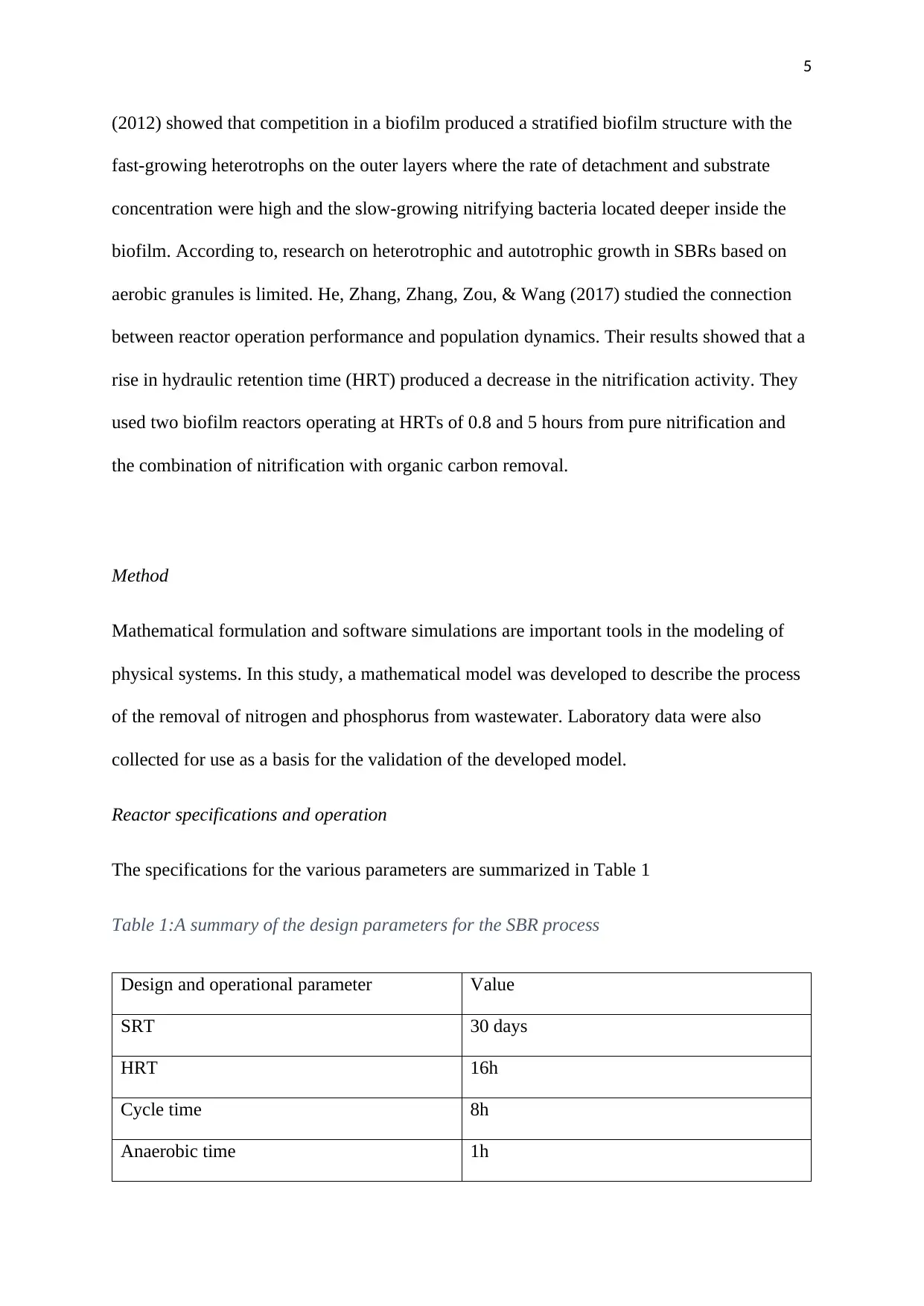
(2012) showed that competition in a biofilm produced a stratified biofilm structure with the
fast-growing heterotrophs on the outer layers where the rate of detachment and substrate
concentration were high and the slow-growing nitrifying bacteria located deeper inside the
biofilm. According to, research on heterotrophic and autotrophic growth in SBRs based on
aerobic granules is limited. He, Zhang, Zhang, Zou, & Wang (2017) studied the connection
between reactor operation performance and population dynamics. Their results showed that a
rise in hydraulic retention time (HRT) produced a decrease in the nitrification activity. They
used two biofilm reactors operating at HRTs of 0.8 and 5 hours from pure nitrification and
the combination of nitrification with organic carbon removal.
Method
Mathematical formulation and software simulations are important tools in the modeling of
physical systems. In this study, a mathematical model was developed to describe the process
of the removal of nitrogen and phosphorus from wastewater. Laboratory data were also
collected for use as a basis for the validation of the developed model.
Reactor specifications and operation
The specifications for the various parameters are summarized in Table 1
Table 1:A summary of the design parameters for the SBR process
Design and operational parameter Value
SRT 30 days
HRT 16h
Cycle time 8h
Anaerobic time 1h
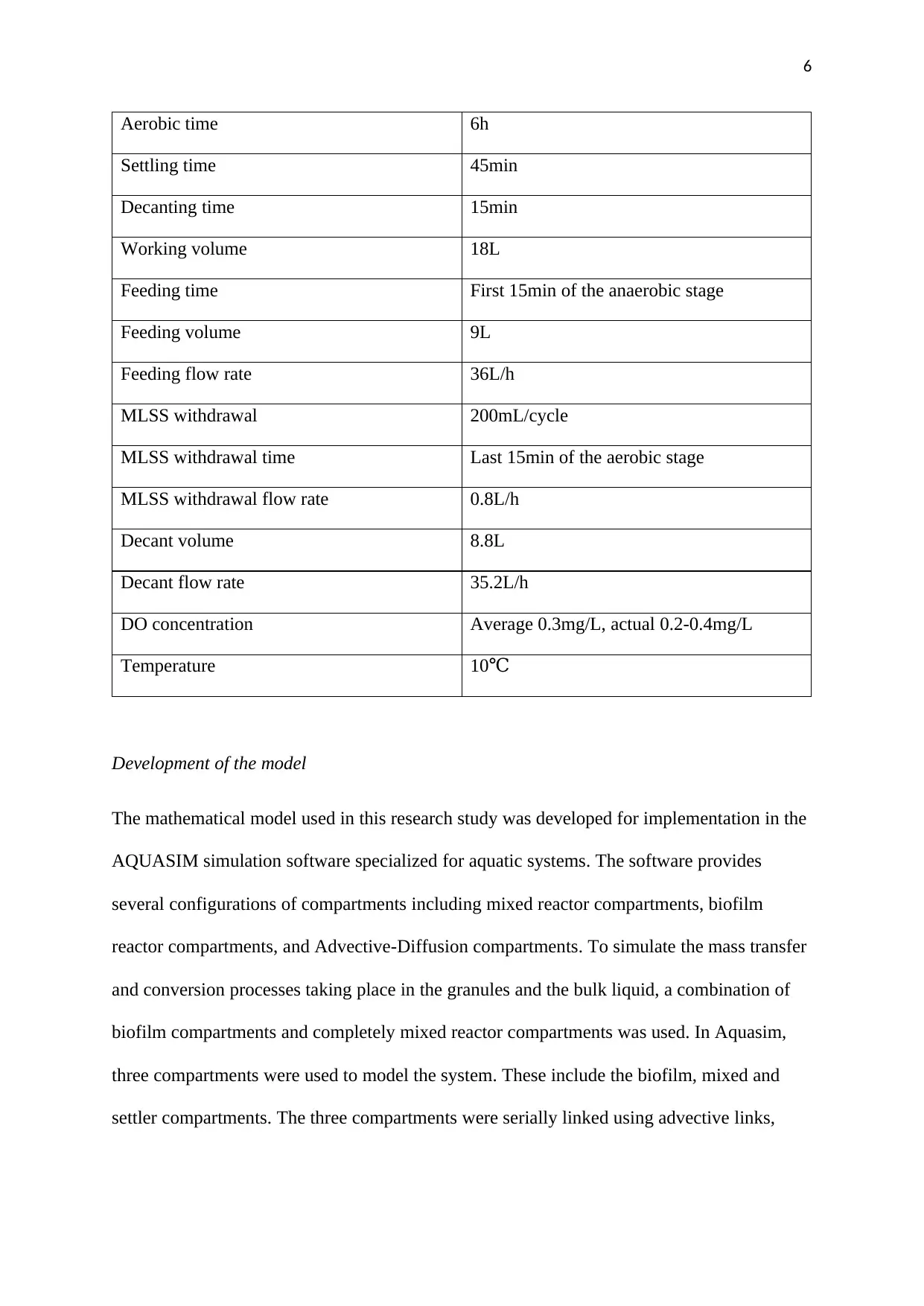
Aerobic time 6h
Settling time 45min
Decanting time 15min
Working volume 18L
Feeding time First 15min of the anaerobic stage
Feeding volume 9L
Feeding flow rate 36L/h
MLSS withdrawal 200mL/cycle
MLSS withdrawal time Last 15min of the aerobic stage
MLSS withdrawal flow rate 0.8L/h
Decant volume 8.8L
Decant flow rate 35.2L/h
DO concentration Average 0.3mg/L, actual 0.2-0.4mg/L
Temperature 10℃
Development of the model
The mathematical model used in this research study was developed for implementation in the
AQUASIM simulation software specialized for aquatic systems. The software provides
several configurations of compartments including mixed reactor compartments, biofilm
reactor compartments, and Advective-Diffusion compartments. To simulate the mass transfer
and conversion processes taking place in the granules and the bulk liquid, a combination of
biofilm compartments and completely mixed reactor compartments was used. In Aquasim,
three compartments were used to model the system. These include the biofilm, mixed and
settler compartments. The three compartments were serially linked using advective links,
⊘ This is a preview!⊘
Do you want full access?
Subscribe today to unlock all pages.

Trusted by 1+ million students worldwide
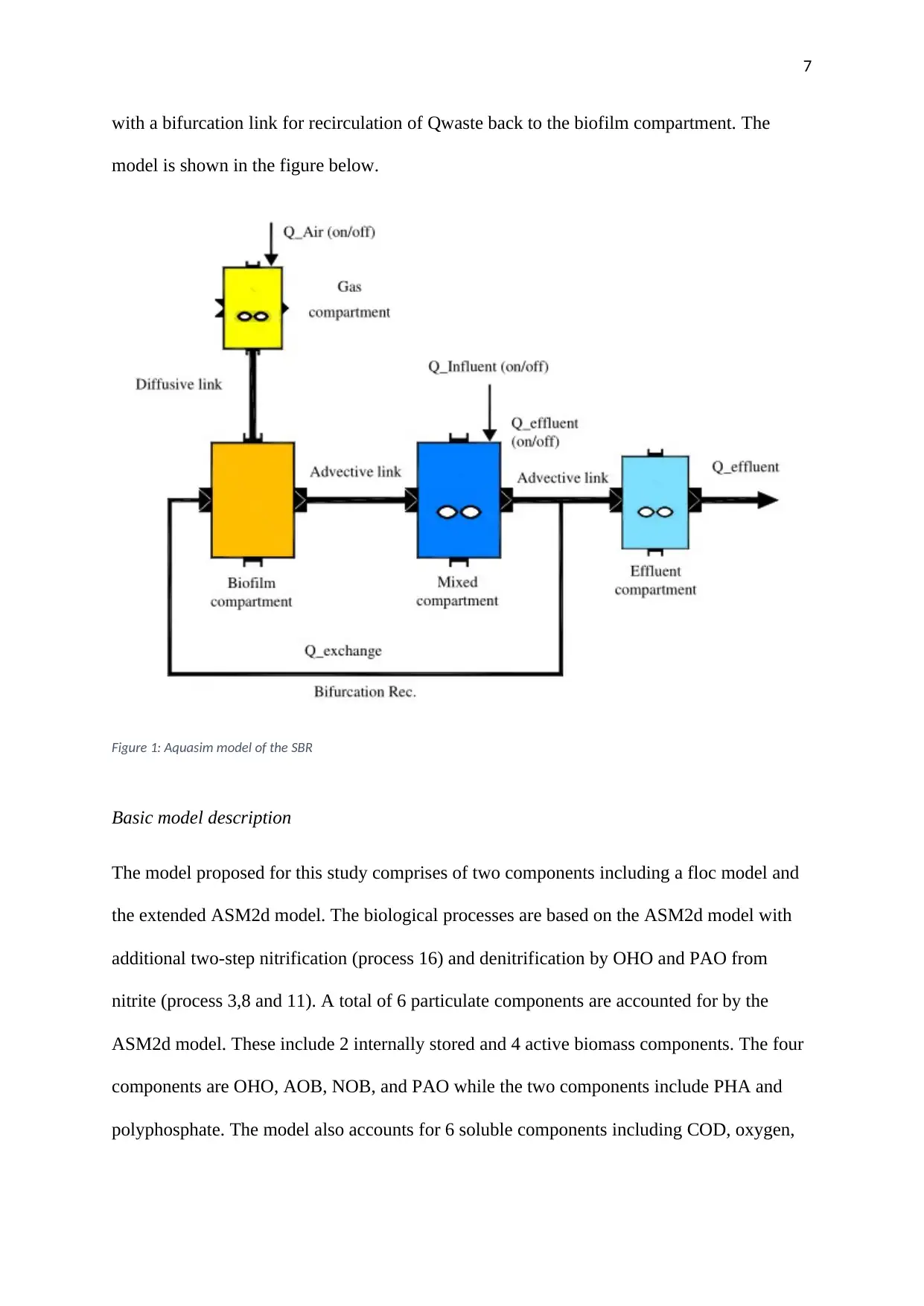
with a bifurcation link for recirculation of Qwaste back to the biofilm compartment. The
model is shown in the figure below.
Figure 1: Aquasim model of the SBR
Basic model description
The model proposed for this study comprises of two components including a floc model and
the extended ASM2d model. The biological processes are based on the ASM2d model with
additional two-step nitrification (process 16) and denitrification by OHO and PAO from
nitrite (process 3,8 and 11). A total of 6 particulate components are accounted for by the
ASM2d model. These include 2 internally stored and 4 active biomass components. The four
components are OHO, AOB, NOB, and PAO while the two components include PHA and
polyphosphate. The model also accounts for 6 soluble components including COD, oxygen,
Paraphrase This Document
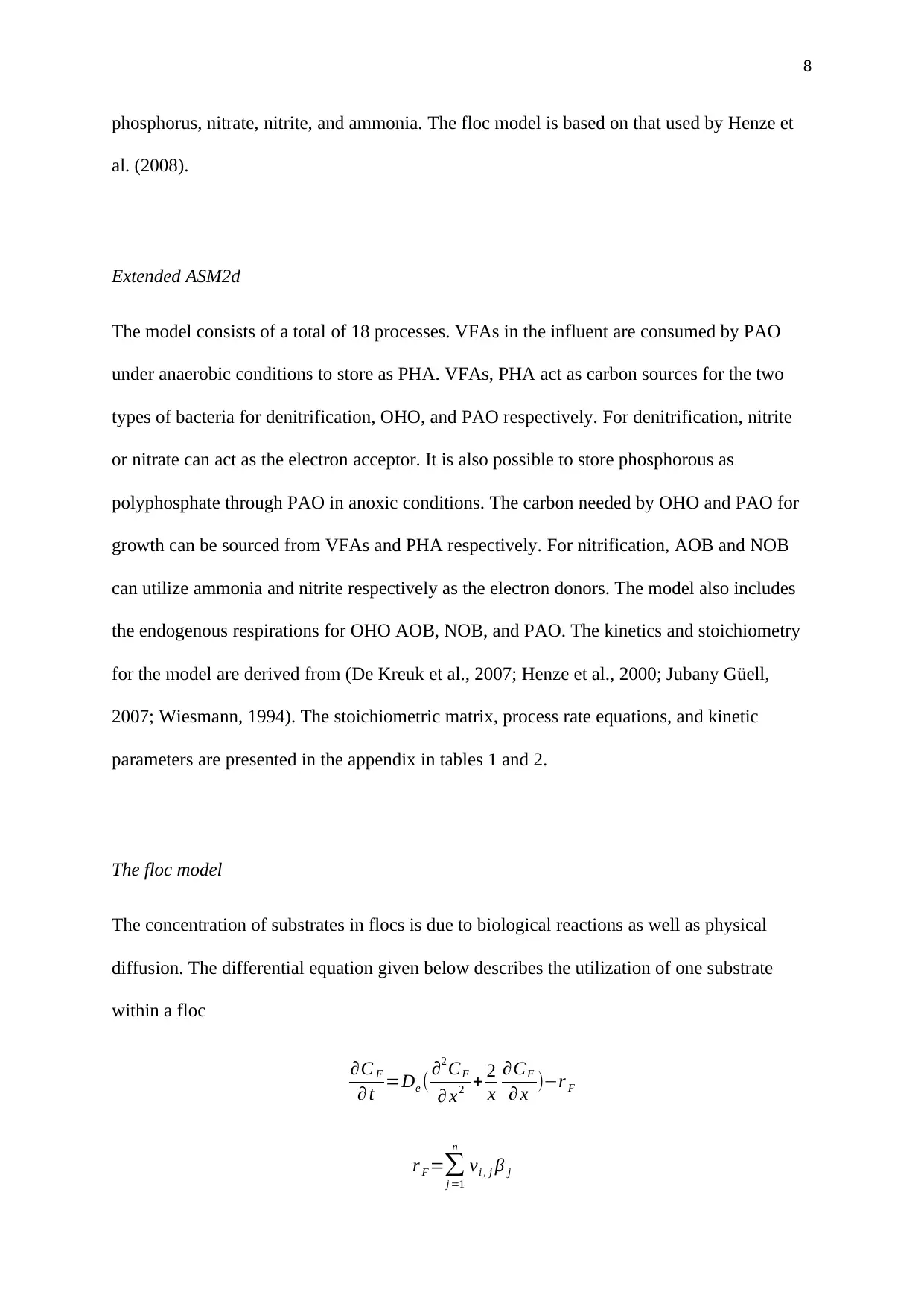
phosphorus, nitrate, nitrite, and ammonia. The floc model is based on that used by Henze et
al. (2008).
Extended ASM2d
The model consists of a total of 18 processes. VFAs in the influent are consumed by PAO
under anaerobic conditions to store as PHA. VFAs, PHA act as carbon sources for the two
types of bacteria for denitrification, OHO, and PAO respectively. For denitrification, nitrite
or nitrate can act as the electron acceptor. It is also possible to store phosphorous as
polyphosphate through PAO in anoxic conditions. The carbon needed by OHO and PAO for
growth can be sourced from VFAs and PHA respectively. For nitrification, AOB and NOB
can utilize ammonia and nitrite respectively as the electron donors. The model also includes
the endogenous respirations for OHO AOB, NOB, and PAO. The kinetics and stoichiometry
for the model are derived from (De Kreuk et al., 2007; Henze et al., 2000; Jubany Güell,
2007; Wiesmann, 1994). The stoichiometric matrix, process rate equations, and kinetic
parameters are presented in the appendix in tables 1 and 2.
The floc model
The concentration of substrates in flocs is due to biological reactions as well as physical
diffusion. The differential equation given below describes the utilization of one substrate
within a floc
∂C F
∂ t =De ( ∂2 CF
∂ x2 + 2
x
∂CF
∂ x )−r F
r F =∑
j =1
n
vi , j β j
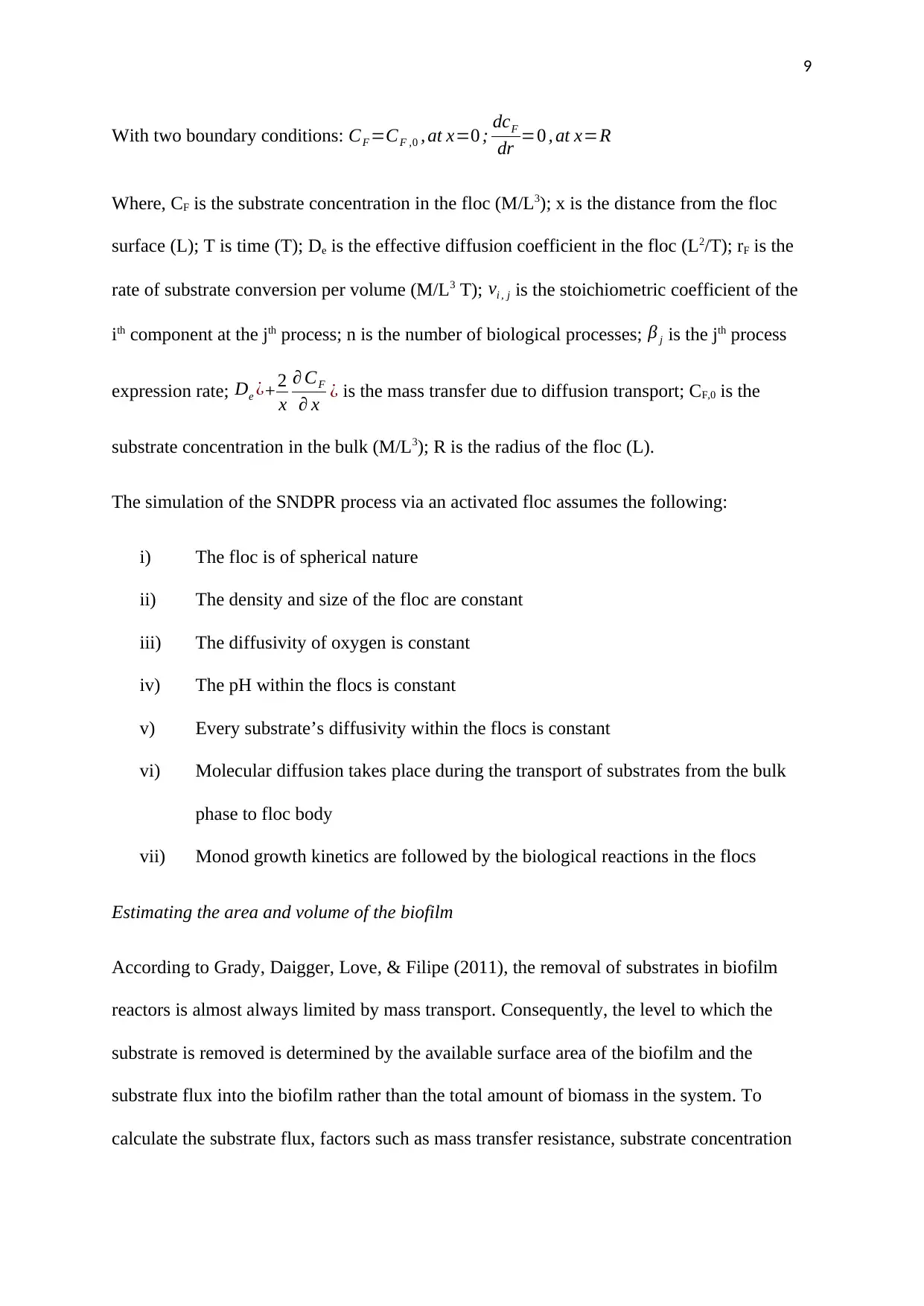
With two boundary conditions: CF =CF ,0 , at x=0 ; dcF
dr =0 , at x=R
Where, CF is the substrate concentration in the floc (M/L3); x is the distance from the floc
surface (L); T is time (T); De is the effective diffusion coefficient in the floc (L2/T); rF is the
rate of substrate conversion per volume (M/L3 T); vi , j is the stoichiometric coefficient of the
ith component at the jth process; n is the number of biological processes; β j is the jth process
expression rate; De ¿+ 2
x
∂ CF
∂ x ¿ is the mass transfer due to diffusion transport; CF,0 is the
substrate concentration in the bulk (M/L3); R is the radius of the floc (L).
The simulation of the SNDPR process via an activated floc assumes the following:
i) The floc is of spherical nature
ii) The density and size of the floc are constant
iii) The diffusivity of oxygen is constant
iv) The pH within the flocs is constant
v) Every substrate’s diffusivity within the flocs is constant
vi) Molecular diffusion takes place during the transport of substrates from the bulk
phase to floc body
vii) Monod growth kinetics are followed by the biological reactions in the flocs
Estimating the area and volume of the biofilm
According to Grady, Daigger, Love, & Filipe (2011), the removal of substrates in biofilm
reactors is almost always limited by mass transport. Consequently, the level to which the
substrate is removed is determined by the available surface area of the biofilm and the
substrate flux into the biofilm rather than the total amount of biomass in the system. To
calculate the substrate flux, factors such as mass transfer resistance, substrate concentration
⊘ This is a preview!⊘
Do you want full access?
Subscribe today to unlock all pages.

Trusted by 1+ million students worldwide
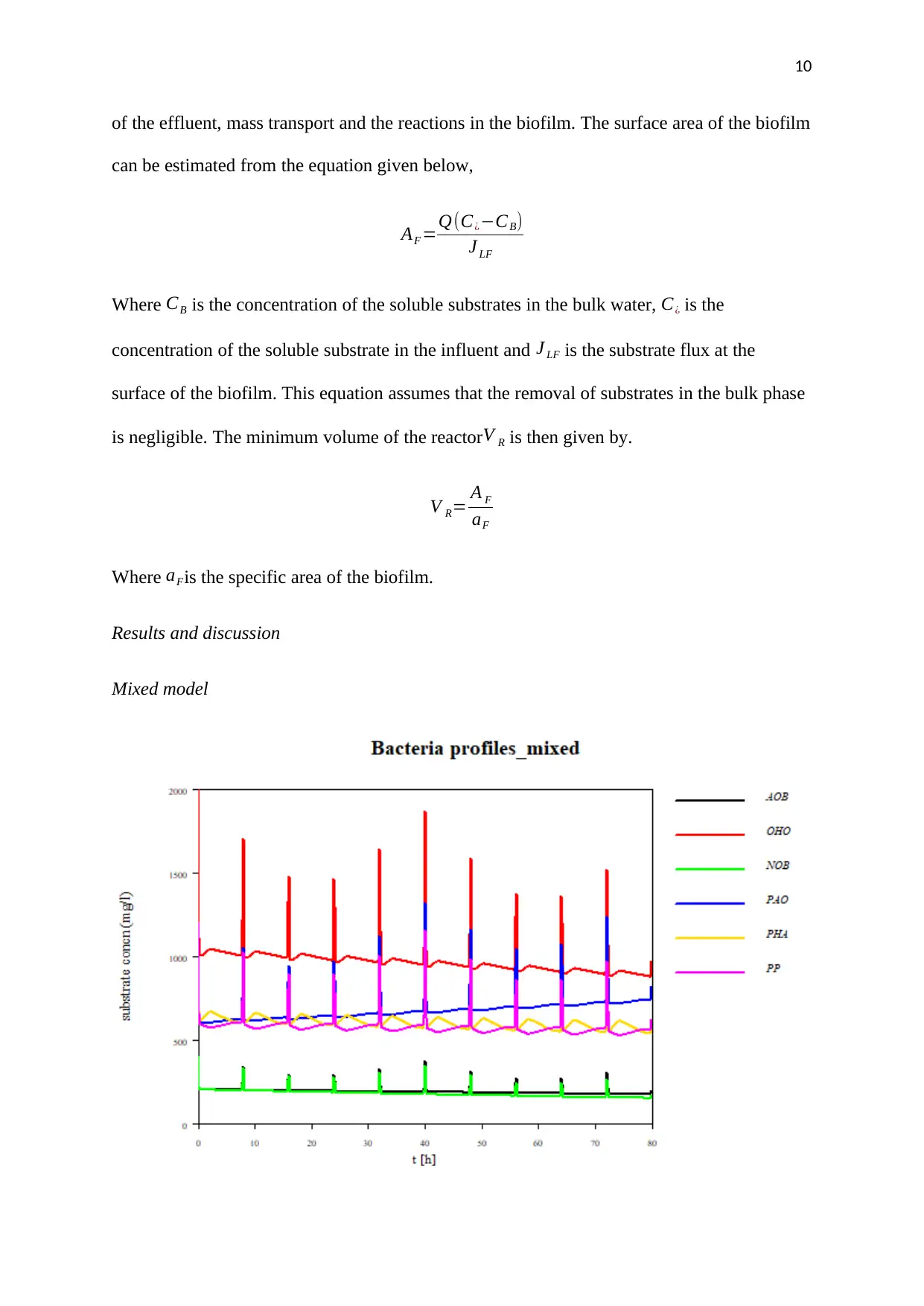
of the effluent, mass transport and the reactions in the biofilm. The surface area of the biofilm
can be estimated from the equation given below,
AF =Q(C¿−CB)
J LF
Where CB is the concentration of the soluble substrates in the bulk water, C¿ is the
concentration of the soluble substrate in the influent and J LF is the substrate flux at the
surface of the biofilm. This equation assumes that the removal of substrates in the bulk phase
is negligible. The minimum volume of the reactorV R is then given by.
V R= A F
aF
Where aFis the specific area of the biofilm.
Results and discussion
Mixed model
Paraphrase This Document
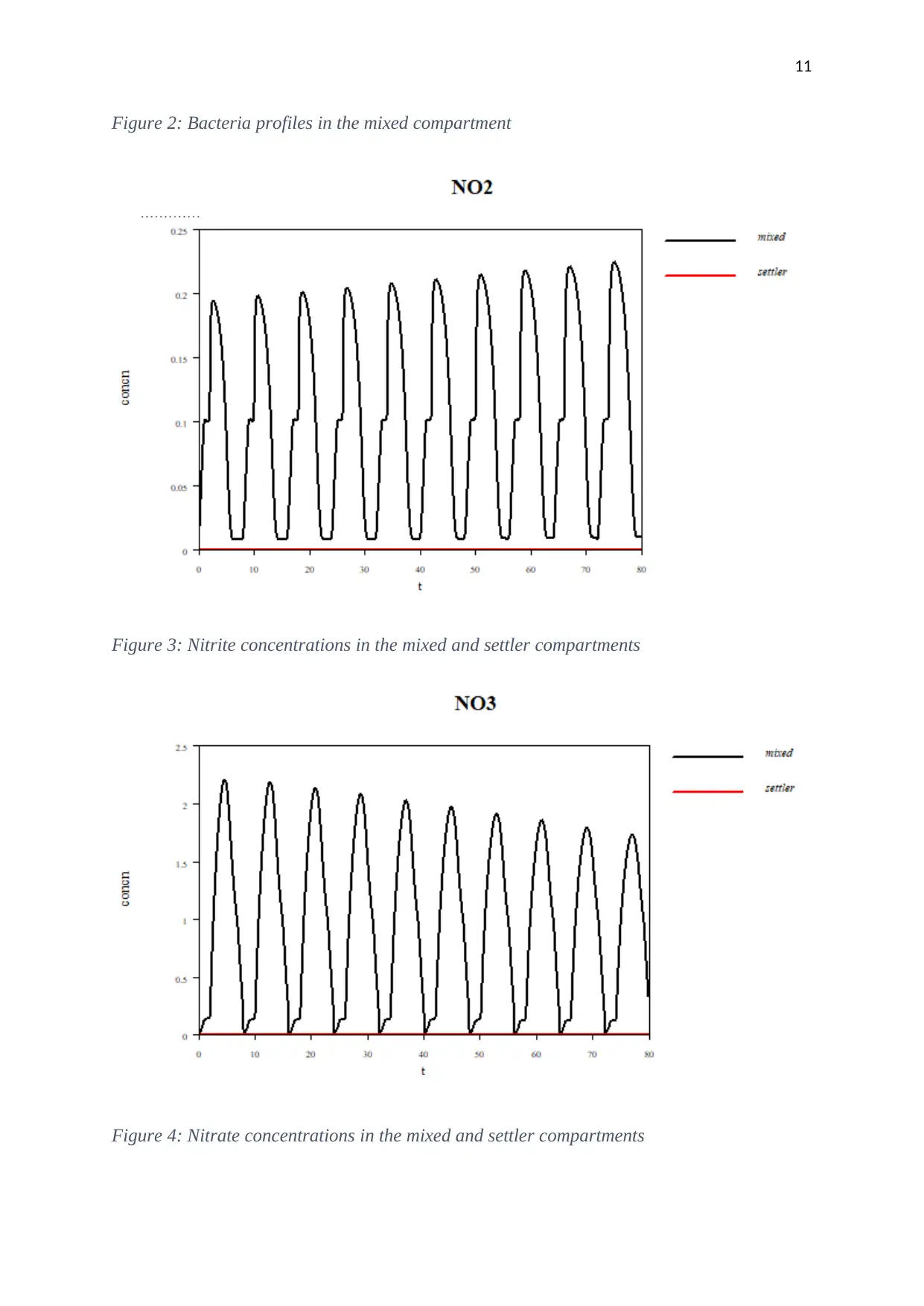
Figure 2: Bacteria profiles in the mixed compartment
Figure 3: Nitrite concentrations in the mixed and settler compartments
Figure 4: Nitrate concentrations in the mixed and settler compartments
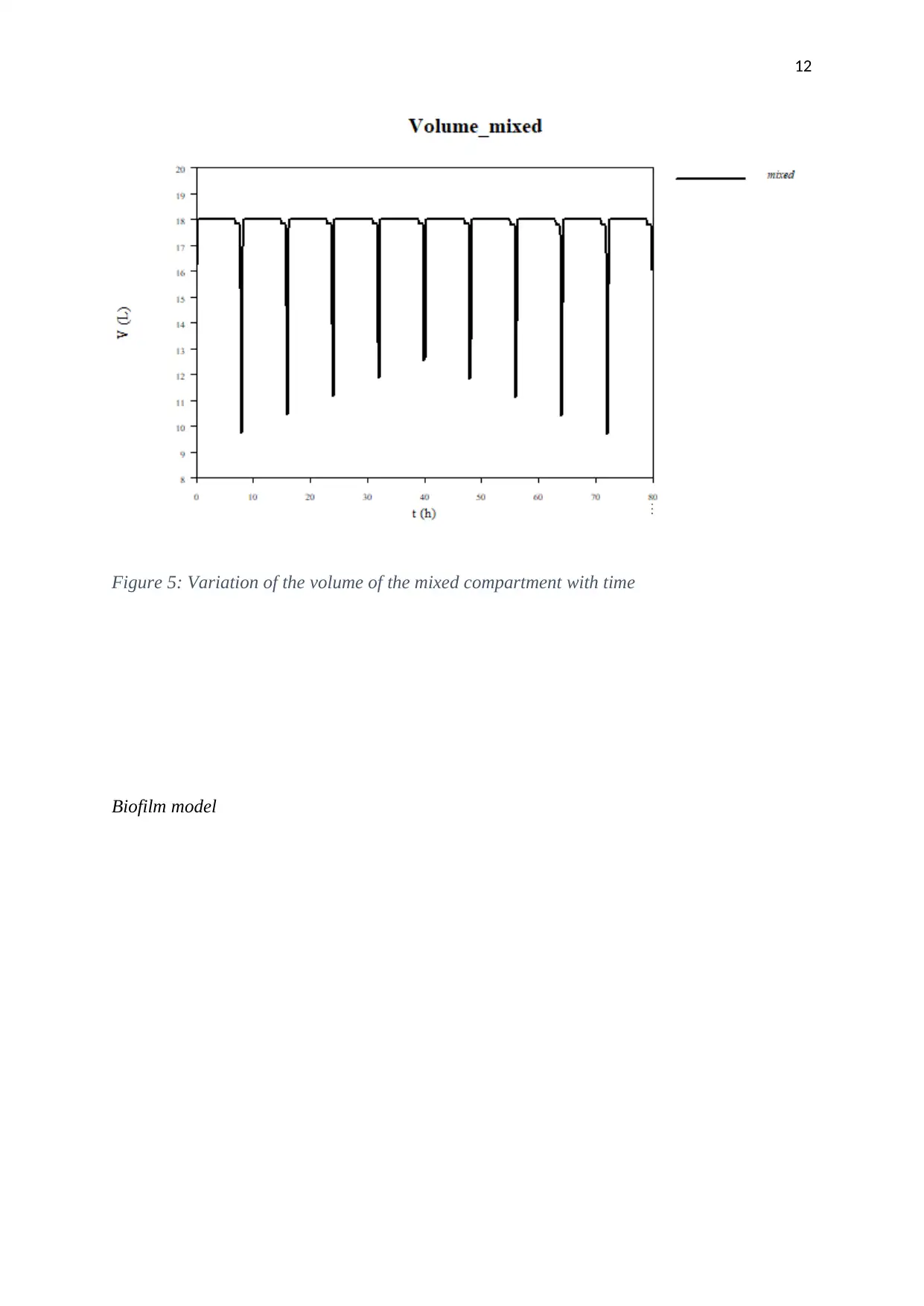
Figure 5: Variation of the volume of the mixed compartment with time
Biofilm model
⊘ This is a preview!⊘
Do you want full access?
Subscribe today to unlock all pages.

Trusted by 1+ million students worldwide
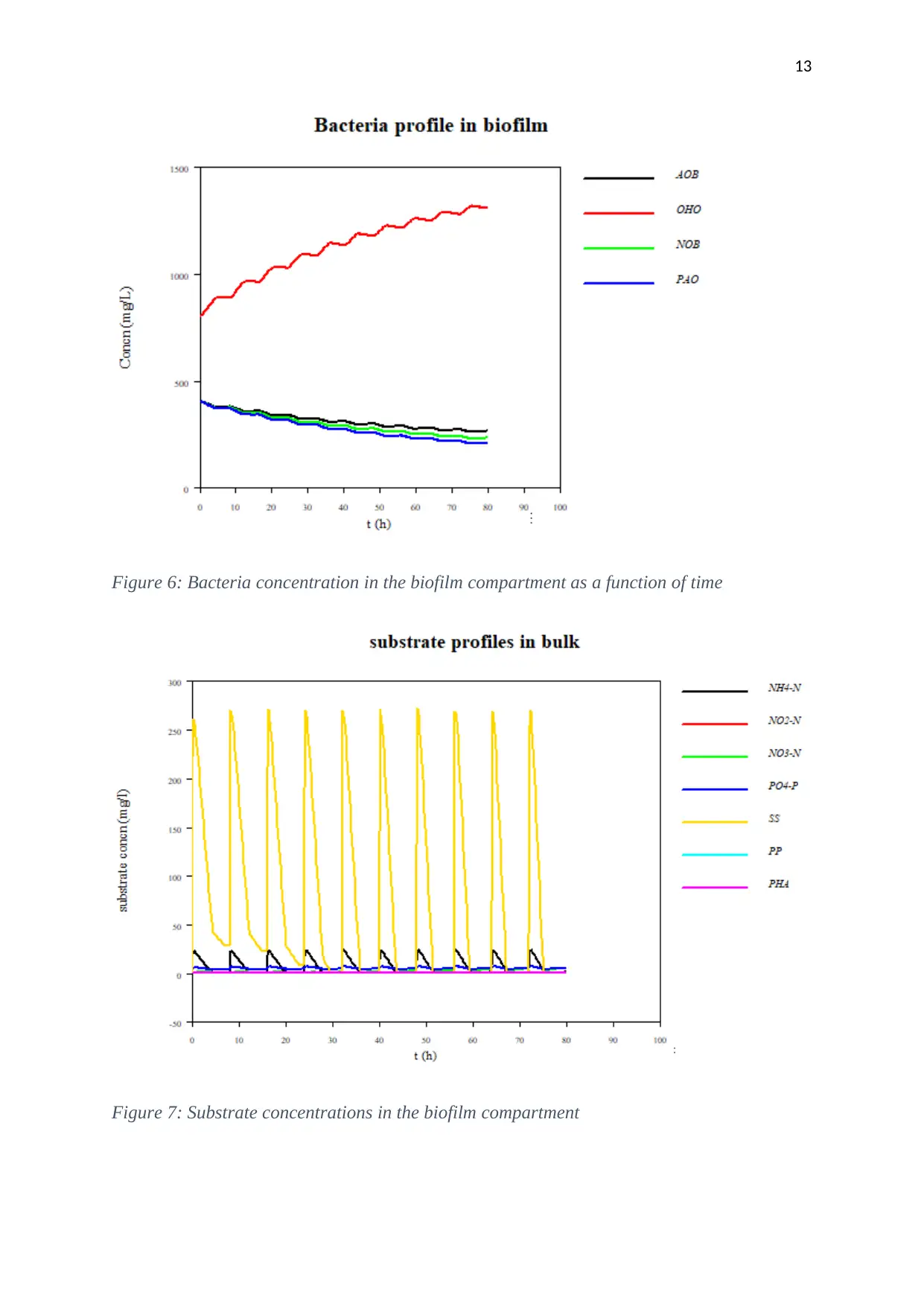
Figure 6: Bacteria concentration in the biofilm compartment as a function of time
Figure 7: Substrate concentrations in the biofilm compartment
Paraphrase This Document
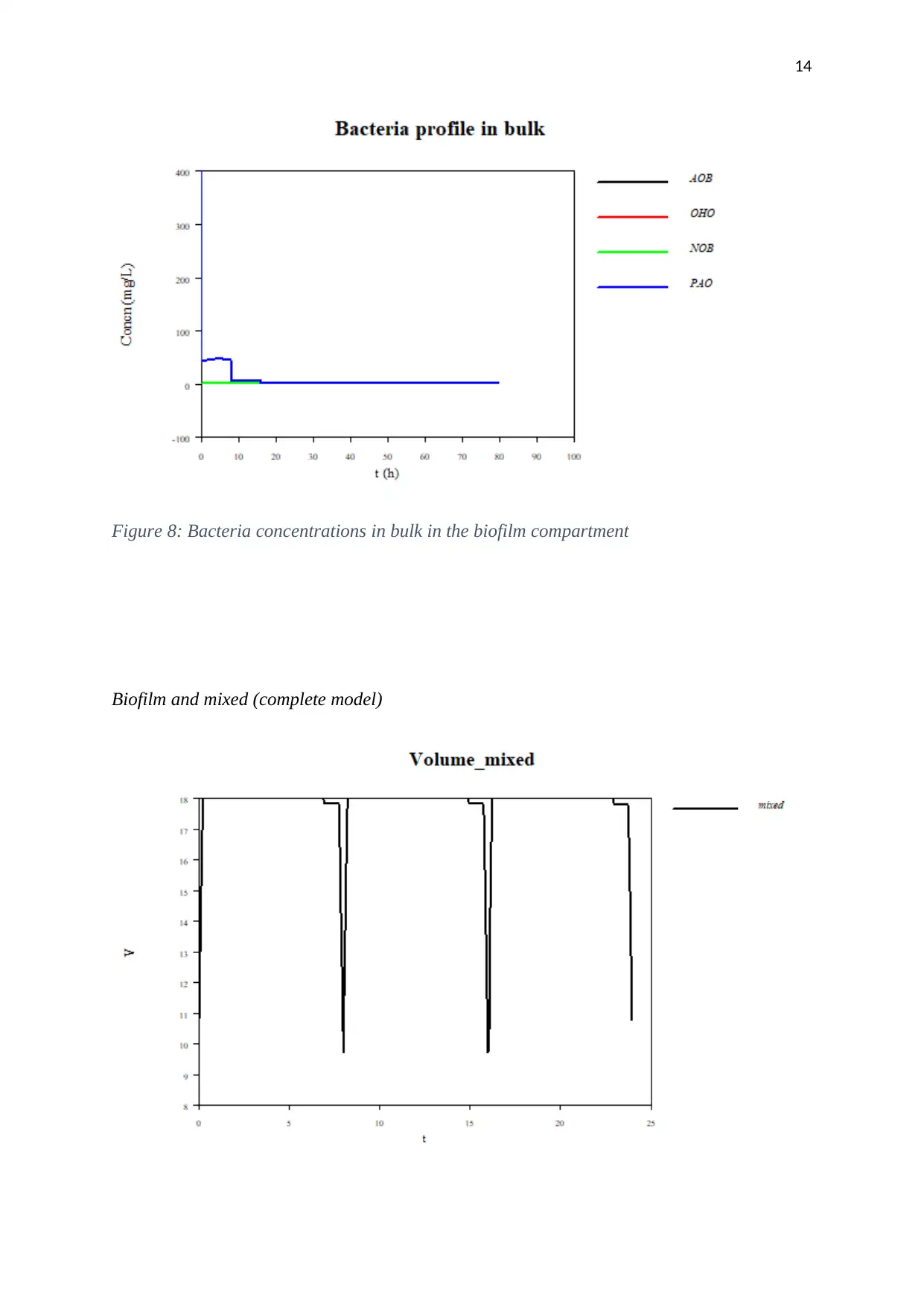
Figure 8: Bacteria concentrations in bulk in the biofilm compartment
Biofilm and mixed (complete model)
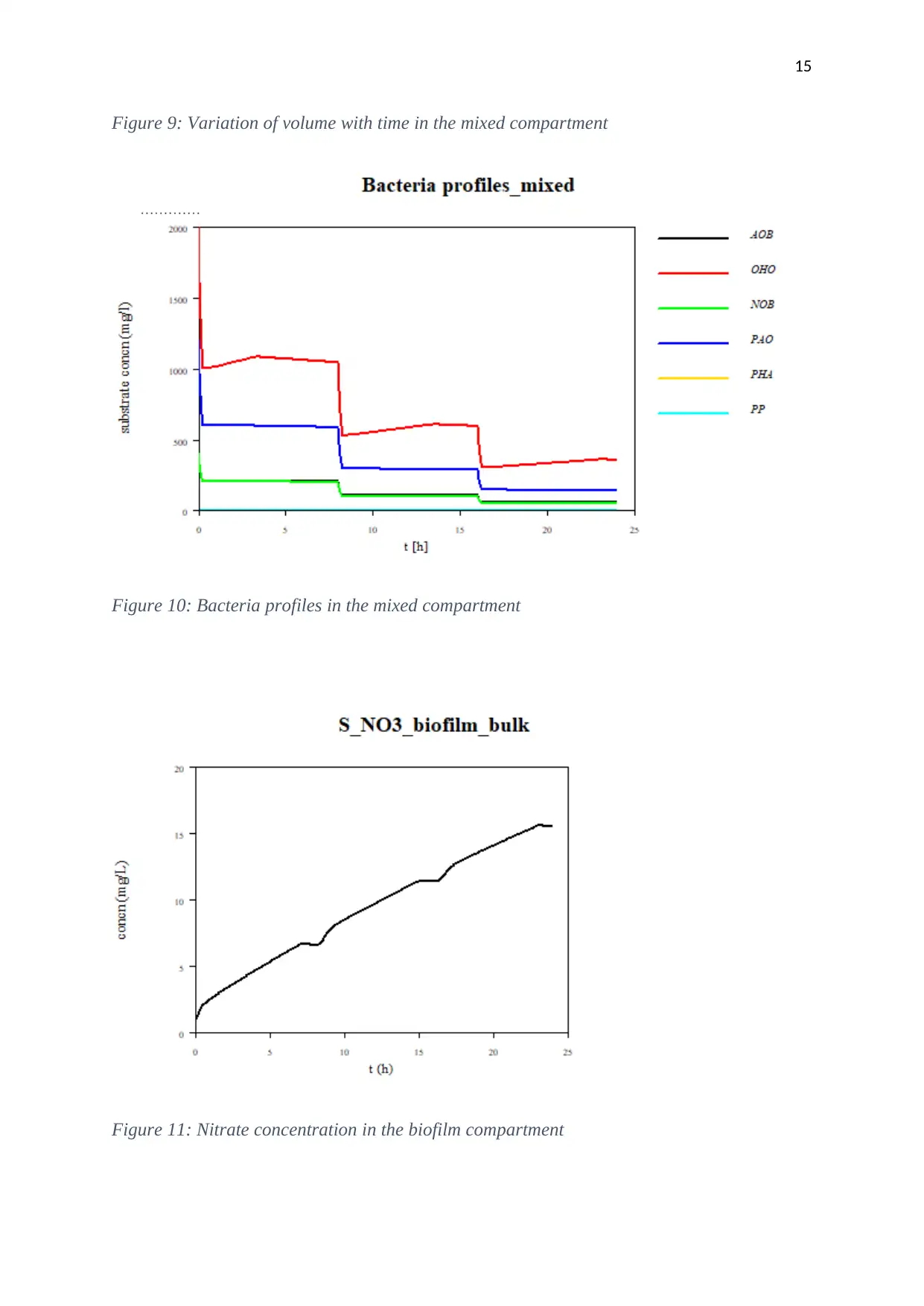
Figure 9: Variation of volume with time in the mixed compartment
Figure 10: Bacteria profiles in the mixed compartment
Figure 11: Nitrate concentration in the biofilm compartment
⊘ This is a preview!⊘
Do you want full access?
Subscribe today to unlock all pages.

Trusted by 1+ million students worldwide
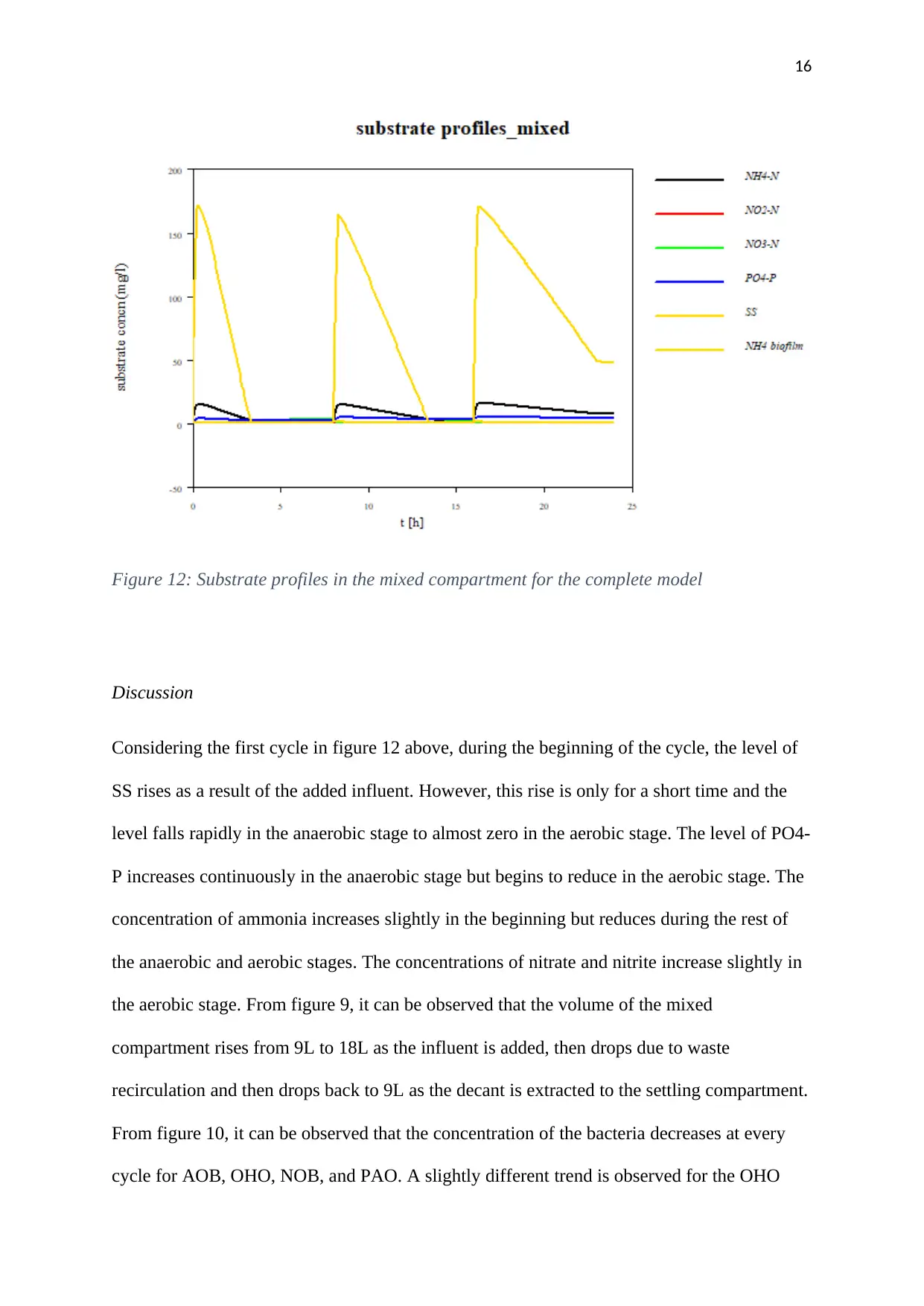
Figure 12: Substrate profiles in the mixed compartment for the complete model
Discussion
Considering the first cycle in figure 12 above, during the beginning of the cycle, the level of
SS rises as a result of the added influent. However, this rise is only for a short time and the
level falls rapidly in the anaerobic stage to almost zero in the aerobic stage. The level of PO4-
P increases continuously in the anaerobic stage but begins to reduce in the aerobic stage. The
concentration of ammonia increases slightly in the beginning but reduces during the rest of
the anaerobic and aerobic stages. The concentrations of nitrate and nitrite increase slightly in
the aerobic stage. From figure 9, it can be observed that the volume of the mixed
compartment rises from 9L to 18L as the influent is added, then drops due to waste
recirculation and then drops back to 9L as the decant is extracted to the settling compartment.
From figure 10, it can be observed that the concentration of the bacteria decreases at every
cycle for AOB, OHO, NOB, and PAO. A slightly different trend is observed for the OHO
Paraphrase This Document
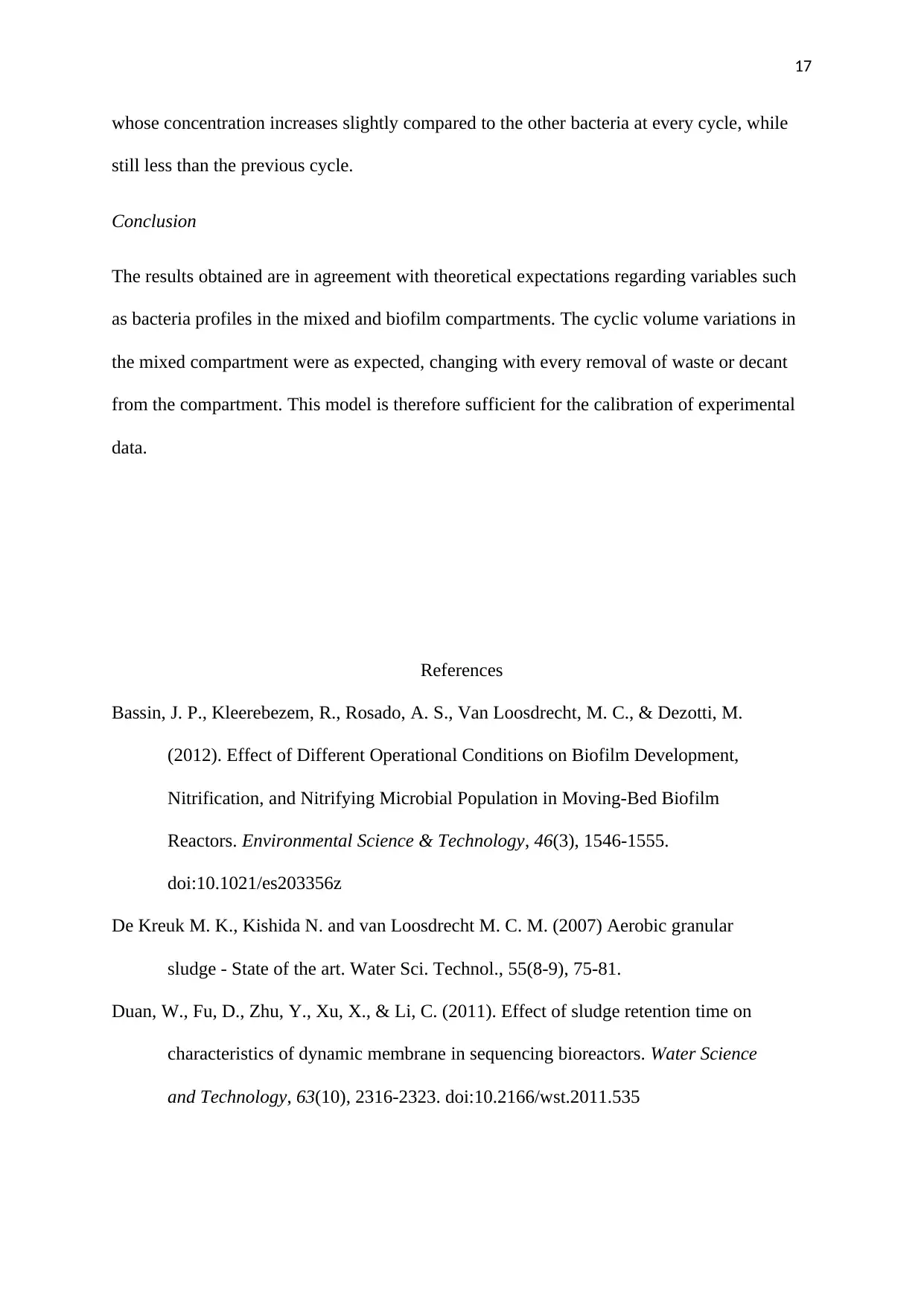
whose concentration increases slightly compared to the other bacteria at every cycle, while
still less than the previous cycle.
Conclusion
The results obtained are in agreement with theoretical expectations regarding variables such
as bacteria profiles in the mixed and biofilm compartments. The cyclic volume variations in
the mixed compartment were as expected, changing with every removal of waste or decant
from the compartment. This model is therefore sufficient for the calibration of experimental
data.
References
Bassin, J. P., Kleerebezem, R., Rosado, A. S., Van Loosdrecht, M. C., & Dezotti, M.
(2012). Effect of Different Operational Conditions on Biofilm Development,
Nitrification, and Nitrifying Microbial Population in Moving-Bed Biofilm
Reactors. Environmental Science & Technology, 46(3), 1546-1555.
doi:10.1021/es203356z
De Kreuk M. K., Kishida N. and van Loosdrecht M. C. M. (2007) Aerobic granular
sludge - State of the art. Water Sci. Technol., 55(8-9), 75-81.
Duan, W., Fu, D., Zhu, Y., Xu, X., & Li, C. (2011). Effect of sludge retention time on
characteristics of dynamic membrane in sequencing bioreactors. Water Science
and Technology, 63(10), 2316-2323. doi:10.2166/wst.2011.535
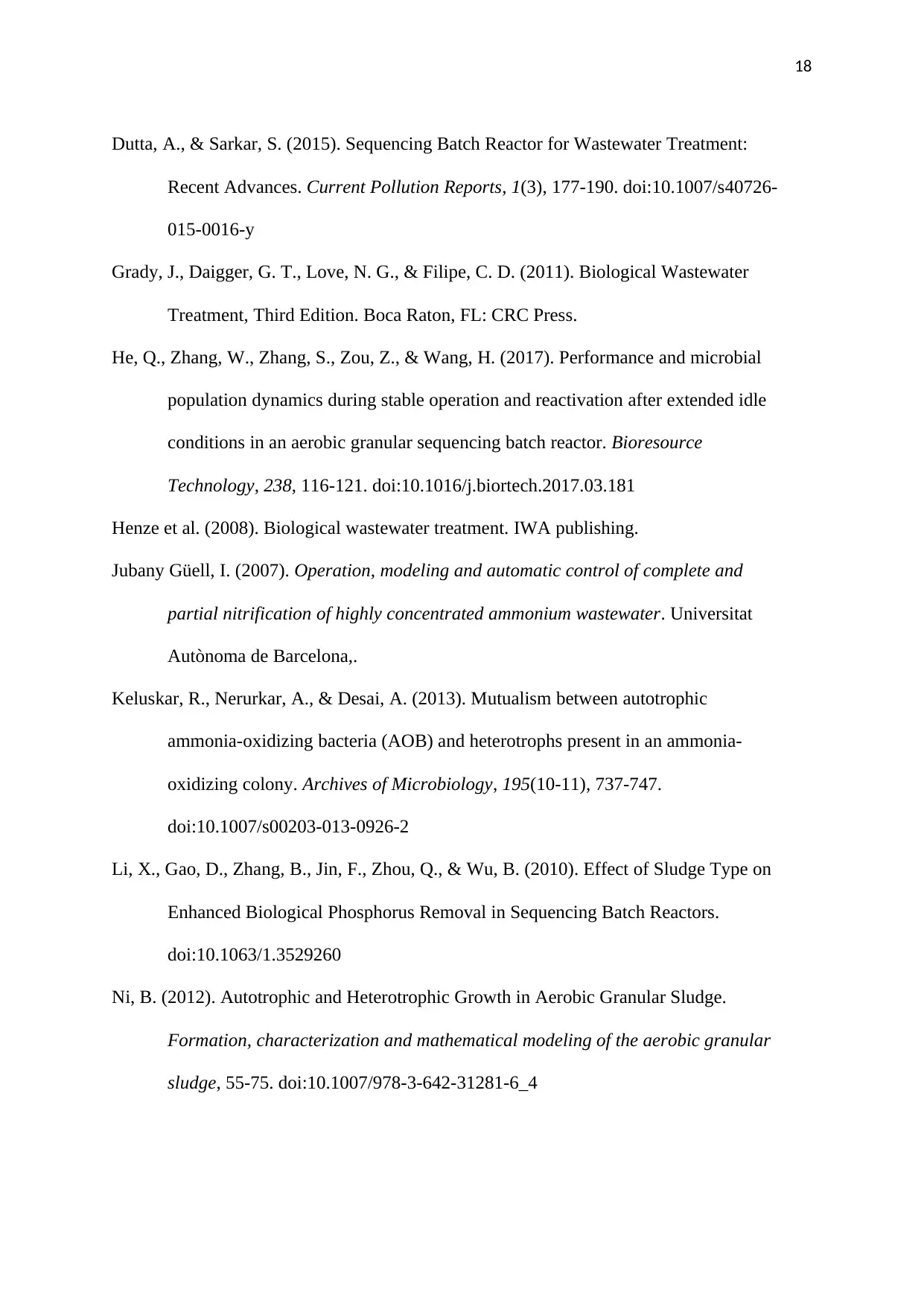
Dutta, A., & Sarkar, S. (2015). Sequencing Batch Reactor for Wastewater Treatment:
Recent Advances. Current Pollution Reports, 1(3), 177-190. doi:10.1007/s40726-
015-0016-y
Grady, J., Daigger, G. T., Love, N. G., & Filipe, C. D. (2011). Biological Wastewater
Treatment, Third Edition. Boca Raton, FL: CRC Press.
He, Q., Zhang, W., Zhang, S., Zou, Z., & Wang, H. (2017). Performance and microbial
population dynamics during stable operation and reactivation after extended idle
conditions in an aerobic granular sequencing batch reactor. Bioresource
Technology, 238, 116-121. doi:10.1016/j.biortech.2017.03.181
Henze et al. (2008). Biological wastewater treatment. IWA publishing.
Jubany Güell, I. (2007). Operation, modeling and automatic control of complete and
partial nitrification of highly concentrated ammonium wastewater. Universitat
Autònoma de Barcelona,.
Keluskar, R., Nerurkar, A., & Desai, A. (2013). Mutualism between autotrophic
ammonia-oxidizing bacteria (AOB) and heterotrophs present in an ammonia-
oxidizing colony. Archives of Microbiology, 195(10-11), 737-747.
doi:10.1007/s00203-013-0926-2
Li, X., Gao, D., Zhang, B., Jin, F., Zhou, Q., & Wu, B. (2010). Effect of Sludge Type on
Enhanced Biological Phosphorus Removal in Sequencing Batch Reactors.
doi:10.1063/1.3529260
Ni, B. (2012). Autotrophic and Heterotrophic Growth in Aerobic Granular Sludge.
Formation, characterization and mathematical modeling of the aerobic granular
sludge, 55-75. doi:10.1007/978-3-642-31281-6_4
⊘ This is a preview!⊘
Do you want full access?
Subscribe today to unlock all pages.

Trusted by 1+ million students worldwide
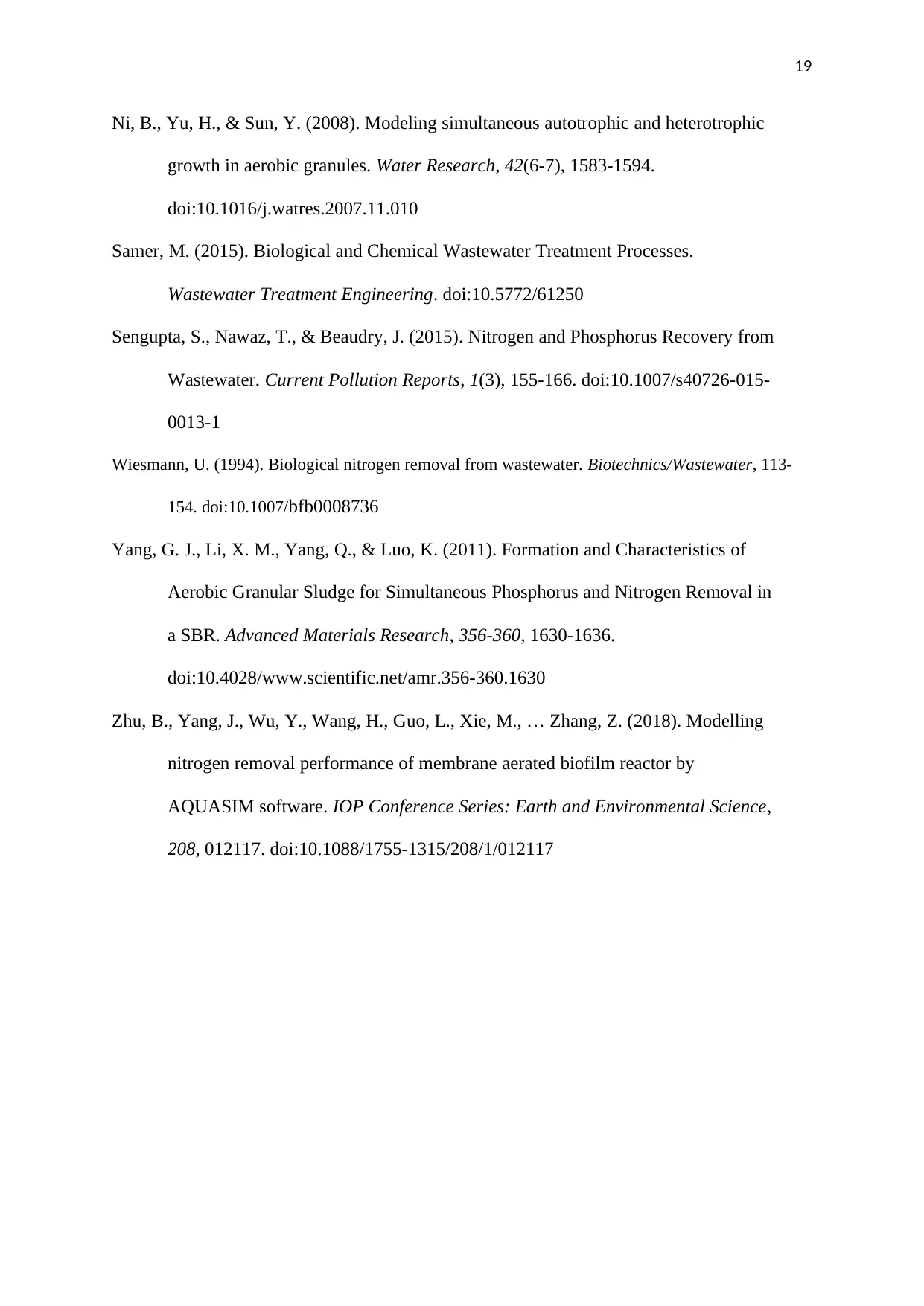
Ni, B., Yu, H., & Sun, Y. (2008). Modeling simultaneous autotrophic and heterotrophic
growth in aerobic granules. Water Research, 42(6-7), 1583-1594.
doi:10.1016/j.watres.2007.11.010
Samer, M. (2015). Biological and Chemical Wastewater Treatment Processes.
Wastewater Treatment Engineering. doi:10.5772/61250
Sengupta, S., Nawaz, T., & Beaudry, J. (2015). Nitrogen and Phosphorus Recovery from
Wastewater. Current Pollution Reports, 1(3), 155-166. doi:10.1007/s40726-015-
0013-1
Wiesmann, U. (1994). Biological nitrogen removal from wastewater. Biotechnics/Wastewater, 113-
154. doi:10.1007/bfb0008736
Yang, G. J., Li, X. M., Yang, Q., & Luo, K. (2011). Formation and Characteristics of
Aerobic Granular Sludge for Simultaneous Phosphorus and Nitrogen Removal in
a SBR. Advanced Materials Research, 356-360, 1630-1636.
doi:10.4028/www.scientific.net/amr.356-360.1630
Zhu, B., Yang, J., Wu, Y., Wang, H., Guo, L., Xie, M., … Zhang, Z. (2018). Modelling
nitrogen removal performance of membrane aerated biofilm reactor by
AQUASIM software. IOP Conference Series: Earth and Environmental Science,
208, 012117. doi:10.1088/1755-1315/208/1/012117
Paraphrase This Document
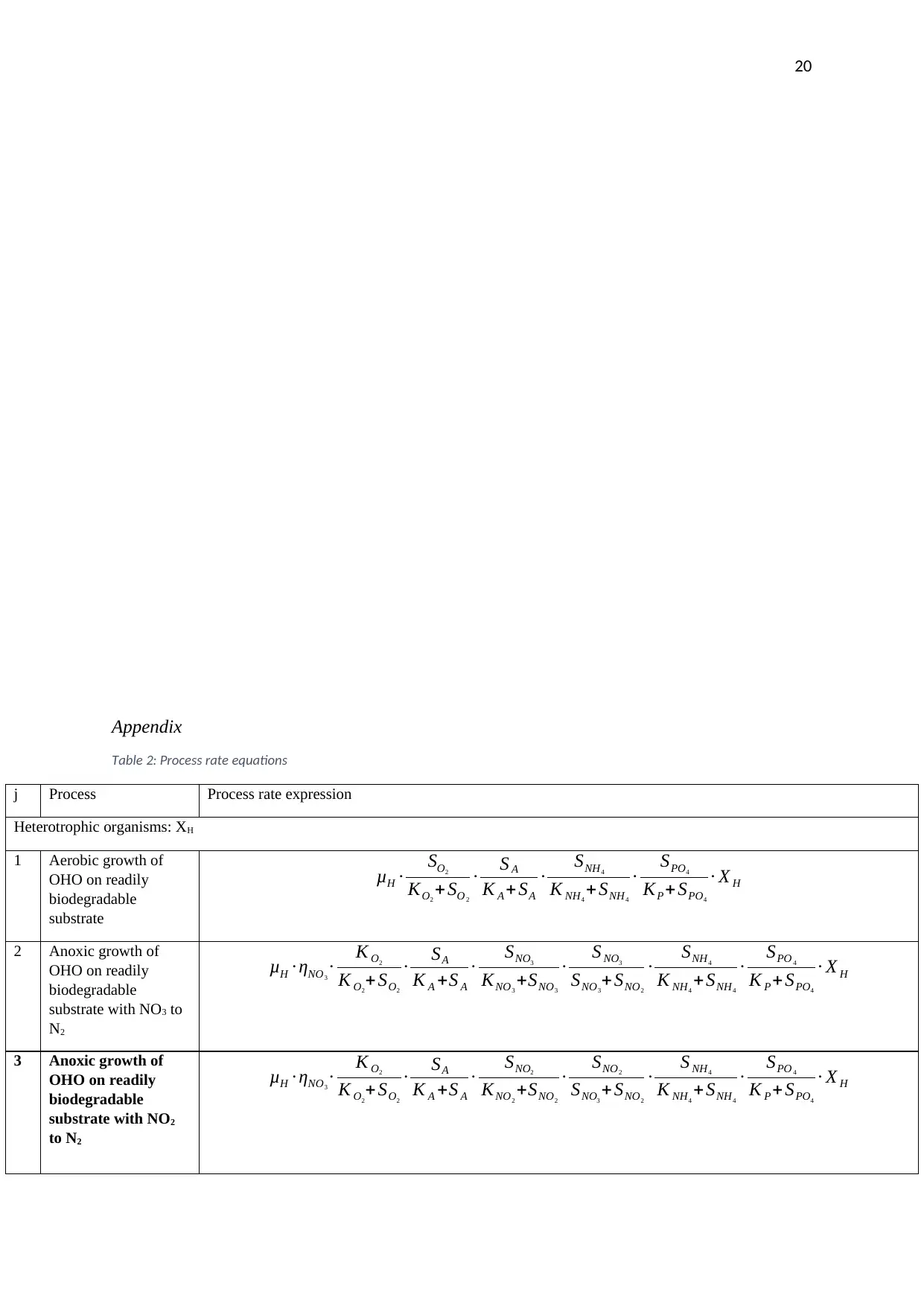
Appendix
Table 2: Process rate equations
j Process Process rate expression
Heterotrophic organisms: XH
1 Aerobic growth of
OHO on readily
biodegradable
substrate
μH ∙ SO2
KO2
+ SO 2
∙ S A
K A +SA
∙ SNH4
K NH4
+ SNH4
∙ SPO4
KP + SPO4
∙ X H
2 Anoxic growth of
OHO on readily
biodegradable
substrate with NO3 to
N2
μH ∙ ƞNO3
∙ K O2
K O2
+ SO2
∙ SA
K A +S A
∙ SNO3
KNO3
+SNO3
∙ S NO3
SNO3
+ SNO2
∙ SNH4
K NH4
+SNH4
∙ SPO 4
K P + SPO4
∙ X H
3 Anoxic growth of
OHO on readily
biodegradable
substrate with NO2
to N2
μH ∙ ƞNO3
∙ K O2
K O2
+ SO2
∙ SA
K A +S A
∙ SNO2
KNO2
+SNO2
∙ SNO2
SNO3
+ SNO2
∙ S NH4
K NH4
+ SNH4
∙ SPO 4
K P + SPO4
∙ X H
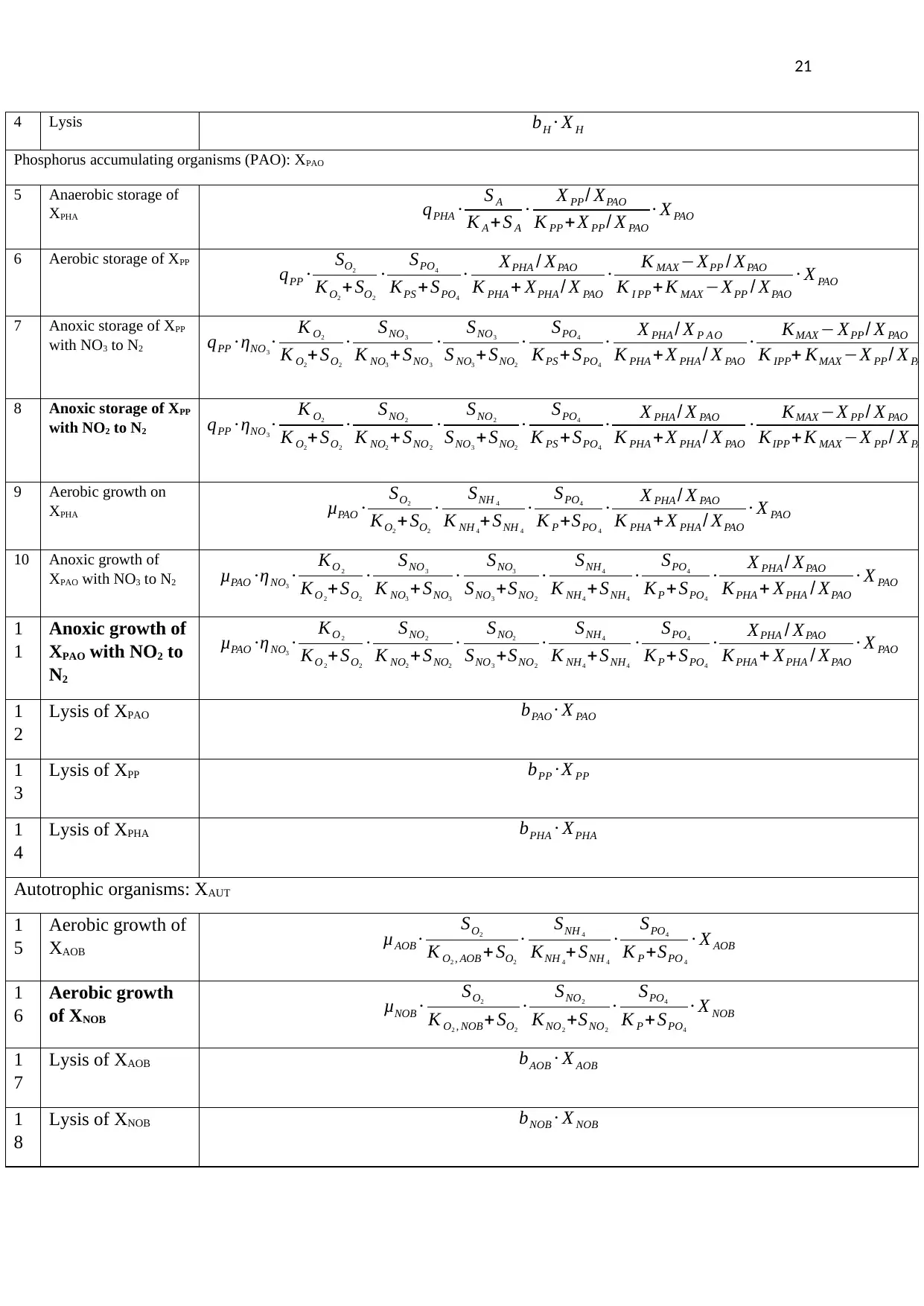
4 Lysis bH ∙ X H
Phosphorus accumulating organisms (PAO): XPAO
5 Anaerobic storage of
XPHA qPHA ∙ S A
K A + S A
∙ X PP / XPAO
K PP + X PP / X PAO
∙ X PAO
6 Aerobic storage of XPP
qPP ∙ SO2
KO2
+ SO2
∙ SPO4
KPS + SPO4
∙ XPHA / XPAO
K PHA + XPHA / X PAO
∙ K MAX −XPP / XPAO
K I PP + K MAX −XPP / XPAO
∙ X PAO
7 Anoxic storage of XPP
with NO3 to N2 qPP ∙ ƞNO3
∙ K O2
K O2
+SO2
∙ SNO3
K NO3
+ SNO3
∙ SNO3
S NO3
+ SNO2
∙ SPO4
KPS + SPO4
∙ X PHA / X P A O
K PHA + X PHA / X PAO
∙ KMAX − XPP / X PAO
K IPP+ KMAX −X PP / X PAO
8 Anoxic storage of XPP
with NO2 to N2 qPP ∙ ƞNO3
∙ K O2
K O2
+SO2
∙ SNO2
K NO2
+ SNO2
∙ SNO2
SNO3
+SNO2
∙ SPO4
K PS +SPO4
∙ X PHA / X PAO
K PHA + X PHA / X PAO
∙ KMAX −X PP / X PAO
KIPP +K MAX −X PP / X PAO
9 Aerobic growth on
XPHA μPAO ∙ SO2
KO2
+ SO2
∙ SNH 4
K NH 4
+ SNH 4
∙ SPO4
K P +SPO 4
∙ X PHA / X PAO
K PHA + X PHA / XPAO
∙ X PAO
10 Anoxic growth of
XPAO with NO3 to N2 μPAO ∙ƞNO3
∙ KO 2
KO 2
+ SO2
∙ SNO3
K NO3
+ SNO3
∙ SNO3
SNO3
+SNO2
∙ SNH4
K NH4
+SNH4
∙ SPO4
KP +SPO4
∙ X PHA / XPAO
KPHA + XPHA / XPAO
∙ X PAO
1
1
Anoxic growth of
XPAO with NO2 to
N2
μPAO ∙ƞNO3
∙ KO 2
KO 2
+ SO2
∙ SNO2
K NO2
+ SNO2
∙ SNO2
SNO3
+SNO2
∙ SNH4
K NH4
+ SNH4
∙ SPO4
KP + SPO4
∙ XPHA / XPAO
KPHA + XPHA / XPAO
∙ X PAO
1
2
Lysis of XPAO bPAO ∙ X PAO
1
3
Lysis of XPP bPP ∙ X PP
1
4
Lysis of XPHA bPHA ∙ XPHA
Autotrophic organisms: XAUT
1
5
Aerobic growth of
XAOB
μAOB ∙ SO2
K O2 , AOB + SO2
∙ SNH 4
KNH 4
+ SNH 4
∙ SPO4
K P +SPO 4
∙ X AOB
1
6
Aerobic growth
of XNOB μNOB ∙ SO2
K O2 , NOB+SO2
∙ SNO2
KNO2
+SNO2
∙ SPO4
K P + SPO4
∙ X NOB
1
7
Lysis of XAOB bAOB ∙ X AOB
1
8
Lysis of XNOB bNOB ∙ X NOB
⊘ This is a preview!⊘
Do you want full access?
Subscribe today to unlock all pages.

Trusted by 1+ million students worldwide
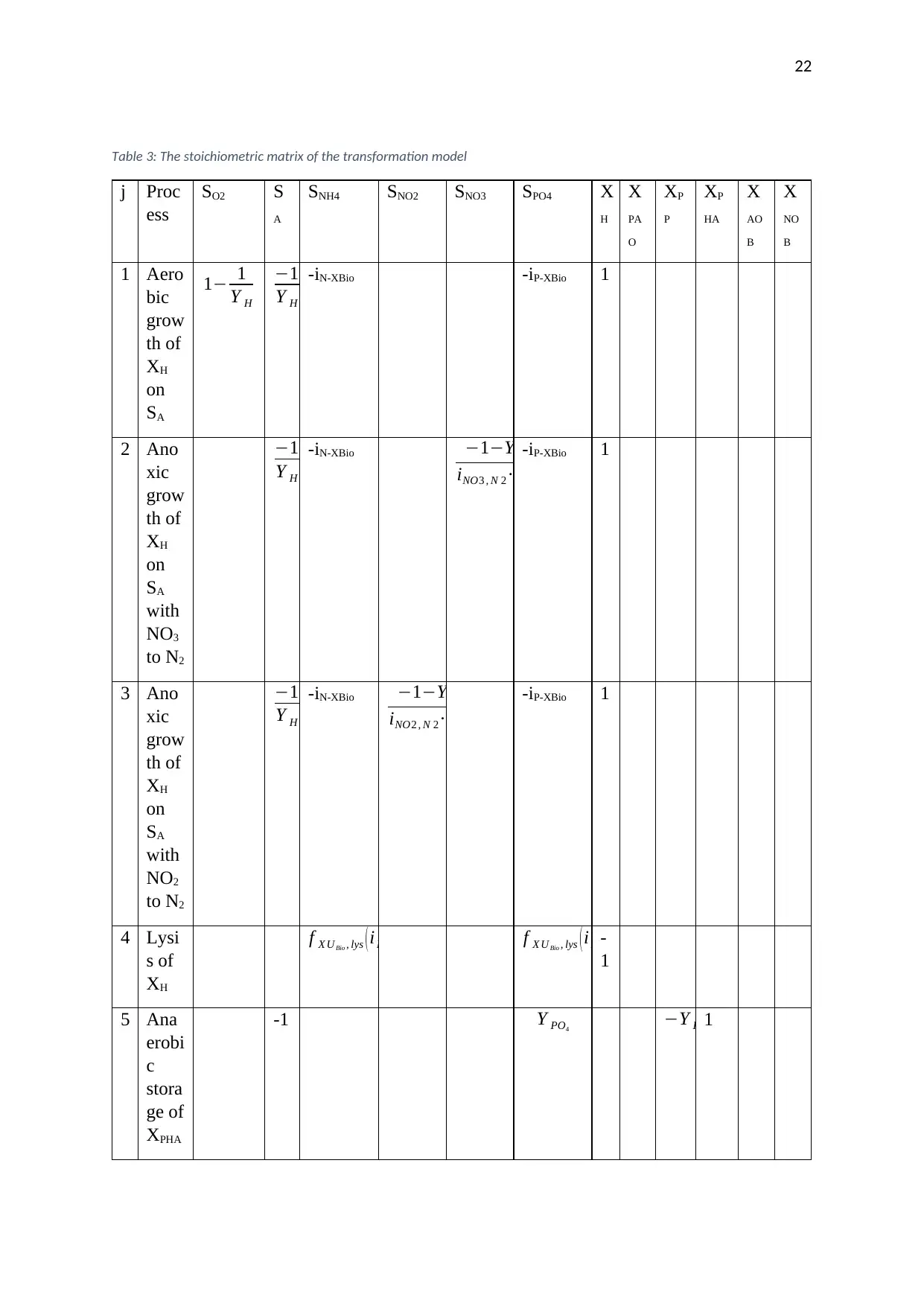
Table 3: The stoichiometric matrix of the transformation model
j Proc
ess
SO2 S
A
SNH4 SNO2 SNO3 SPO4 X
H
X
PA
O
XP
P
XP
HA
X
AO
B
X
NO
B
1 Aero
bic
grow
th of
XH
on
SA
1− 1
Y H
−1
Y H
-iN-XBio -iP-XBio 1
2 Ano
xic
grow
th of
XH
on
SA
with
NO3
to N2
−1
Y H
-iN-XBio −1−Y H
iNO3 , N 2 ∙ Y H
-iP-XBio 1
3 Ano
xic
grow
th of
XH
on
SA
with
NO2
to N2
−1
Y H
-iN-XBio −1−Y H
iNO2 , N 2 ∙ Y H
-iP-XBio 1
4 Lysi
s of
XH
f X U Bio , lys ( iN XCB
−iN XU ) −(iN XCB
−iN XBio
)f X U Bio , lys (iPXCB
−iPXU )−(iP XCB
−iP XBio
)-
1
5 Ana
erobi
c
stora
ge of
XPHA
-1 Y PO4
−Y PO4
1
Paraphrase This Document
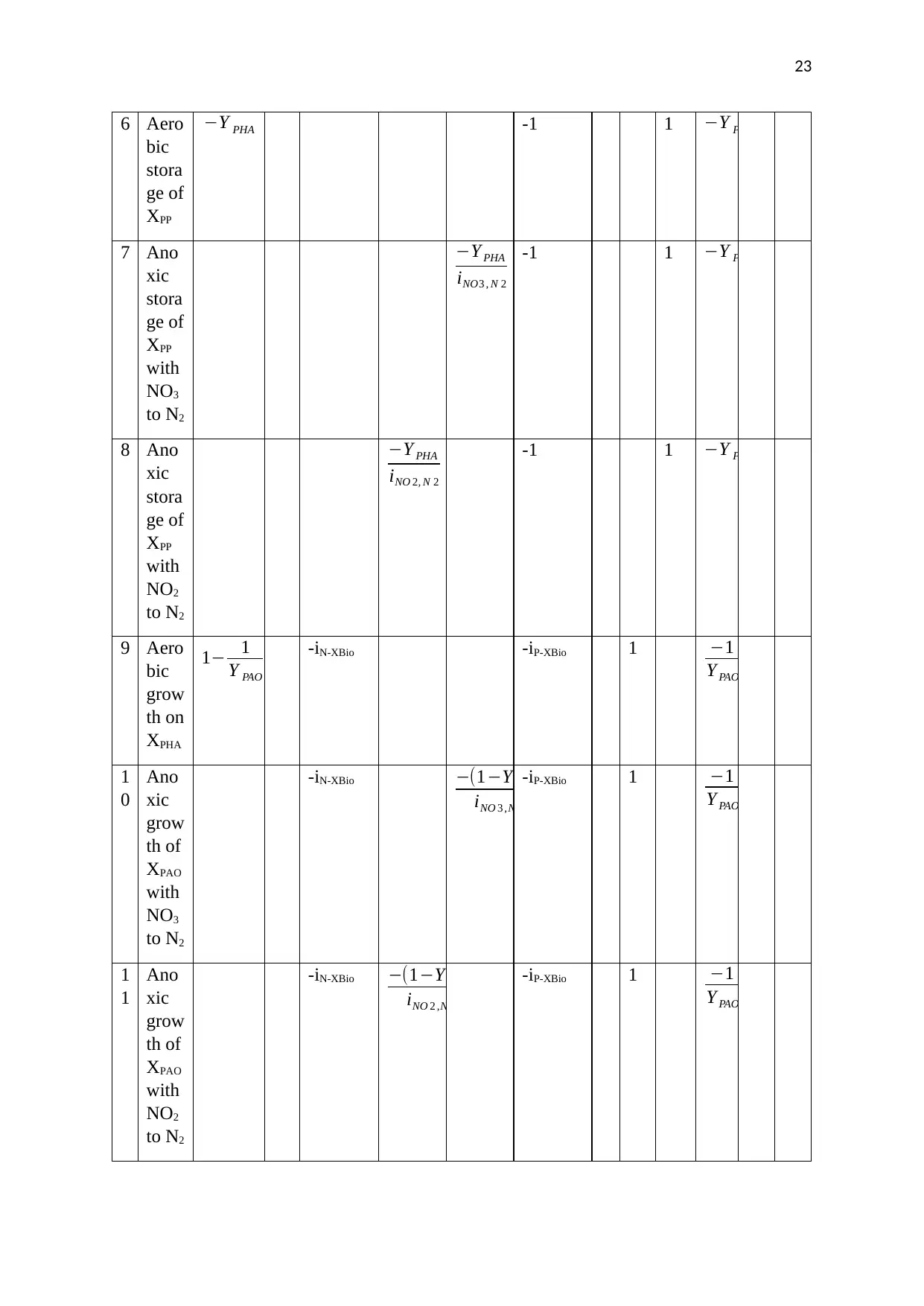
6 Aero
bic
stora
ge of
XPP
−Y PHA -1 1 −Y PHA
7 Ano
xic
stora
ge of
XPP
with
NO3
to N2
−Y PHA
iNO3 , N 2
-1 1 −Y PHA
8 Ano
xic
stora
ge of
XPP
with
NO2
to N2
−Y PHA
iNO 2, N 2
-1 1 −Y PHA
9 Aero
bic
grow
th on
XPHA
1− 1
Y PAO
-iN-XBio -iP-XBio 1 −1
Y PAO
1
0
Ano
xic
grow
th of
XPAO
with
NO3
to N2
-iN-XBio −(1−Y ¿¿ PAO)
iNO 3 ,N 2 Y PAO
¿
-iP-XBio 1 −1
Y PAO
1
1
Ano
xic
grow
th of
XPAO
with
NO2
to N2
-iN-XBio −(1−Y ¿¿ PAO)
iNO 2 ,N 2 Y PAO
¿-iP-XBio 1 −1
Y PAO
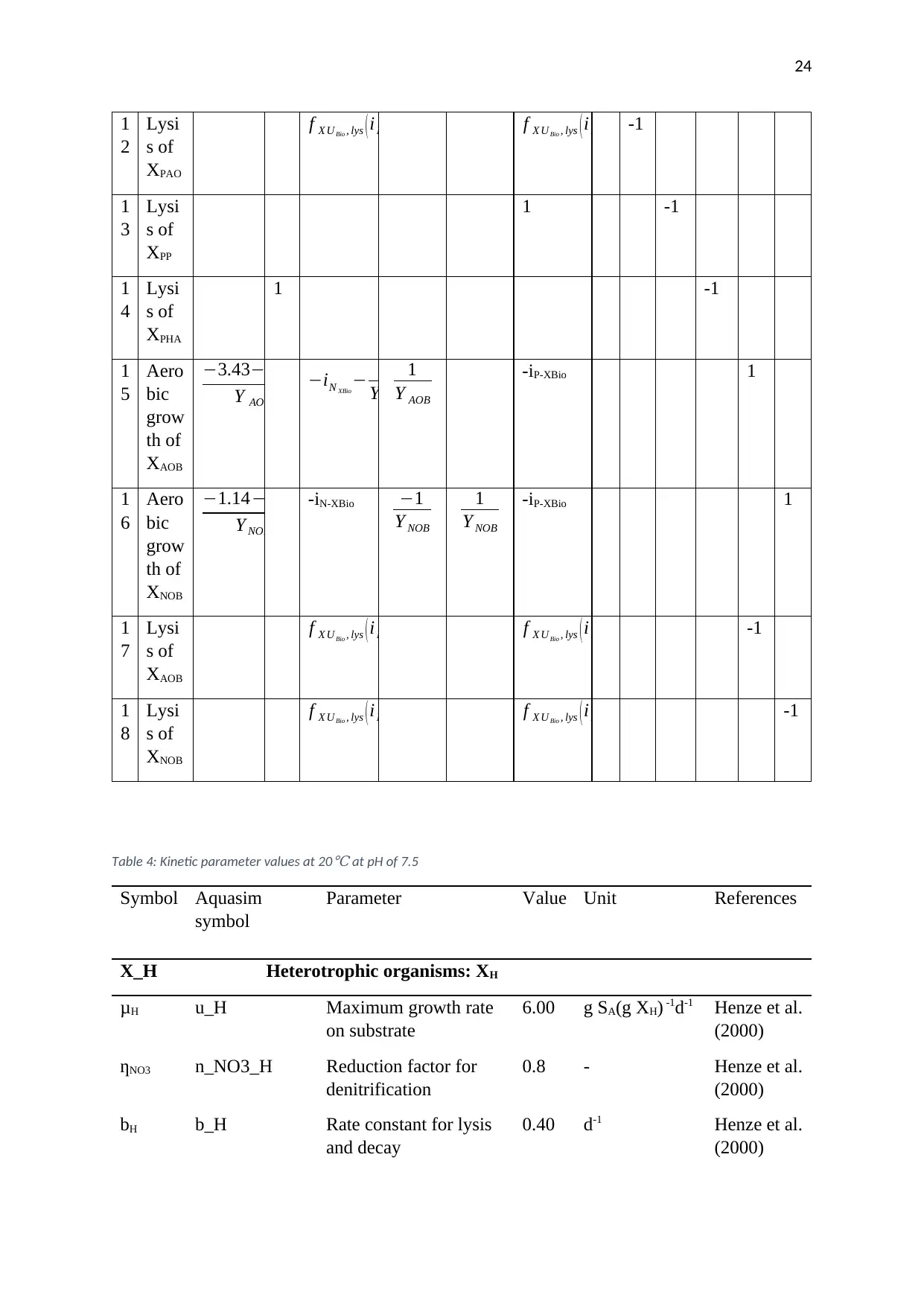
1
2
Lysi
s of
XPAO
f X U Bio , lys ( iN XCB
−iN XU ) −(iN XCB
−iN XBio
)f X U Bio , lys (iPXCB
−iPXU )−(iP XCB
−iP XBio
)-1
1
3
Lysi
s of
XPP
1 -1
1
4
Lysi
s of
XPHA
1 -1
1
5
Aero
bic
grow
th of
XAOB
−3.43−Y AOB
Y AOB
−iN XBio
− 1
Y AOB
1
Y AOB
-iP-XBio 1
1
6
Aero
bic
grow
th of
XNOB
−1.14−Y NOB
Y NOB
-iN-XBio −1
Y NOB
1
Y NOB
-iP-XBio 1
1
7
Lysi
s of
XAOB
f X U Bio , lys ( iN XCB
−iN XU ) −(iN XCB
−iN XBio
)f X U Bio , lys (iPXCB
−iPXU )−(iP XCB
−iP XBio
)-1
1
8
Lysi
s of
XNOB
f X U Bio , lys ( iN XCB
−iN XU ) −(iN XCB
−iN XBio
)f X U Bio , lys (iPXCB
−iPXU )−(iP XCB
−iP XBio
) -1
Table 4: Kinetic parameter values at 20
℃ at pH of 7.5
Symbol Aquasim
symbol
Parameter Value Unit References
X_H Heterotrophic organisms: XH
μH u_H Maximum growth rate
on substrate
6.00 g SA(g XH) -1d-1 Henze et al.
(2000)
ÆžNO3 n_NO3_H Reduction factor for
denitrification
0.8 - Henze et al.
(2000)
bH b_H Rate constant for lysis
and decay
0.40 d-1 Henze et al.
(2000)
⊘ This is a preview!⊘
Do you want full access?
Subscribe today to unlock all pages.

Trusted by 1+ million students worldwide
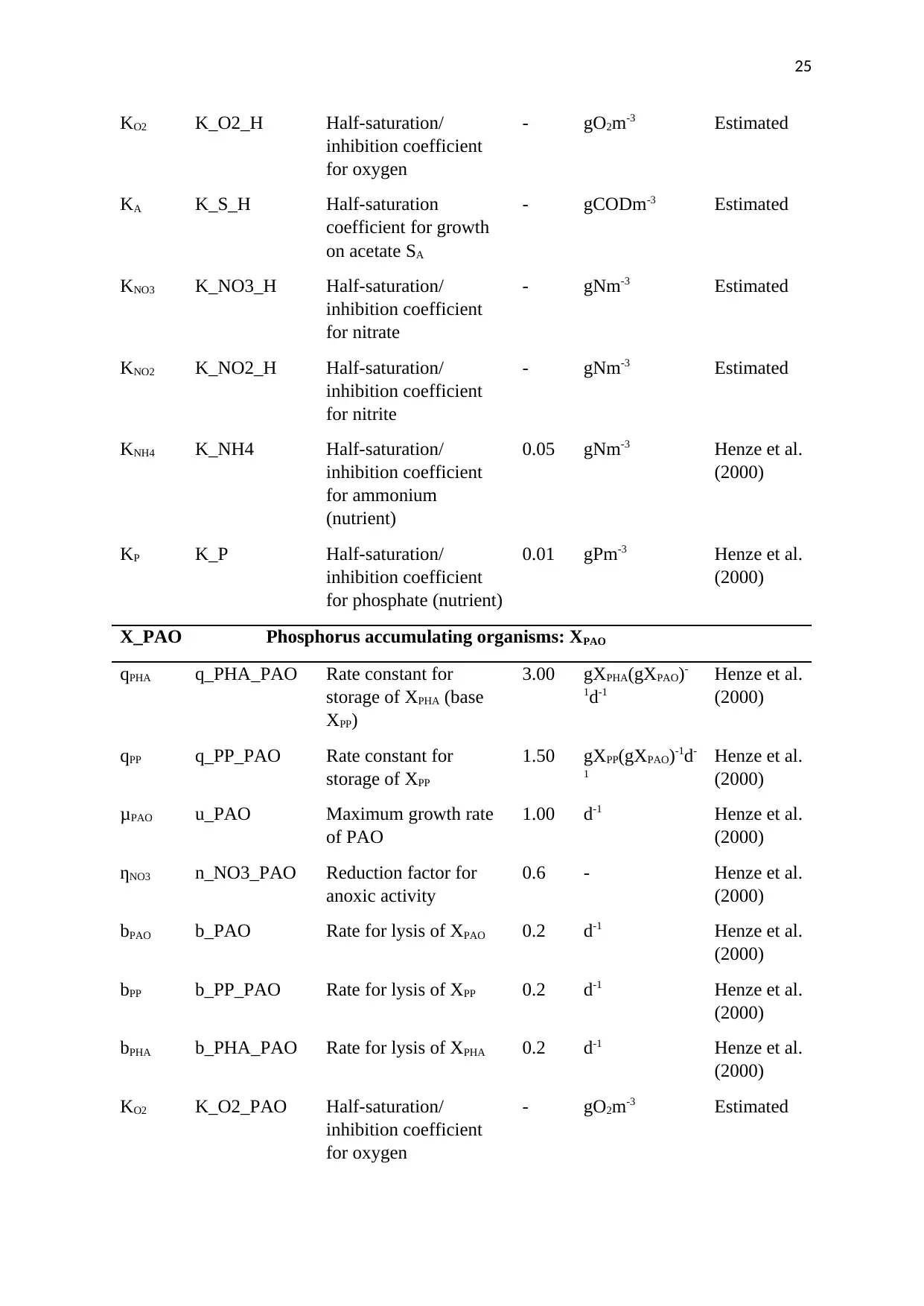
KO2 K_O2_H Half-saturation/
inhibition coefficient
for oxygen
- gO2m-3 Estimated
KA K_S_H Half-saturation
coefficient for growth
on acetate SA
- gCODm-3 Estimated
KNO3 K_NO3_H Half-saturation/
inhibition coefficient
for nitrate
- gNm-3 Estimated
KNO2 K_NO2_H Half-saturation/
inhibition coefficient
for nitrite
- gNm-3 Estimated
KNH4 K_NH4 Half-saturation/
inhibition coefficient
for ammonium
(nutrient)
0.05 gNm-3 Henze et al.
(2000)
KP K_P Half-saturation/
inhibition coefficient
for phosphate (nutrient)
0.01 gPm-3 Henze et al.
(2000)
X_PAO Phosphorus accumulating organisms: XPAO
qPHA q_PHA_PAO Rate constant for
storage of XPHA (base
XPP)
3.00 gXPHA(gXPAO)-
1d-1
Henze et al.
(2000)
qPP q_PP_PAO Rate constant for
storage of XPP
1.50 gXPP(gXPAO)-1d-
1
Henze et al.
(2000)
μPAO u_PAO Maximum growth rate
of PAO
1.00 d-1 Henze et al.
(2000)
ÆžNO3 n_NO3_PAO Reduction factor for
anoxic activity
0.6 - Henze et al.
(2000)
bPAO b_PAO Rate for lysis of XPAO 0.2 d-1 Henze et al.
(2000)
bPP b_PP_PAO Rate for lysis of XPP 0.2 d-1 Henze et al.
(2000)
bPHA b_PHA_PAO Rate for lysis of XPHA 0.2 d-1 Henze et al.
(2000)
KO2 K_O2_PAO Half-saturation/
inhibition coefficient
for oxygen
- gO2m-3 Estimated
Paraphrase This Document
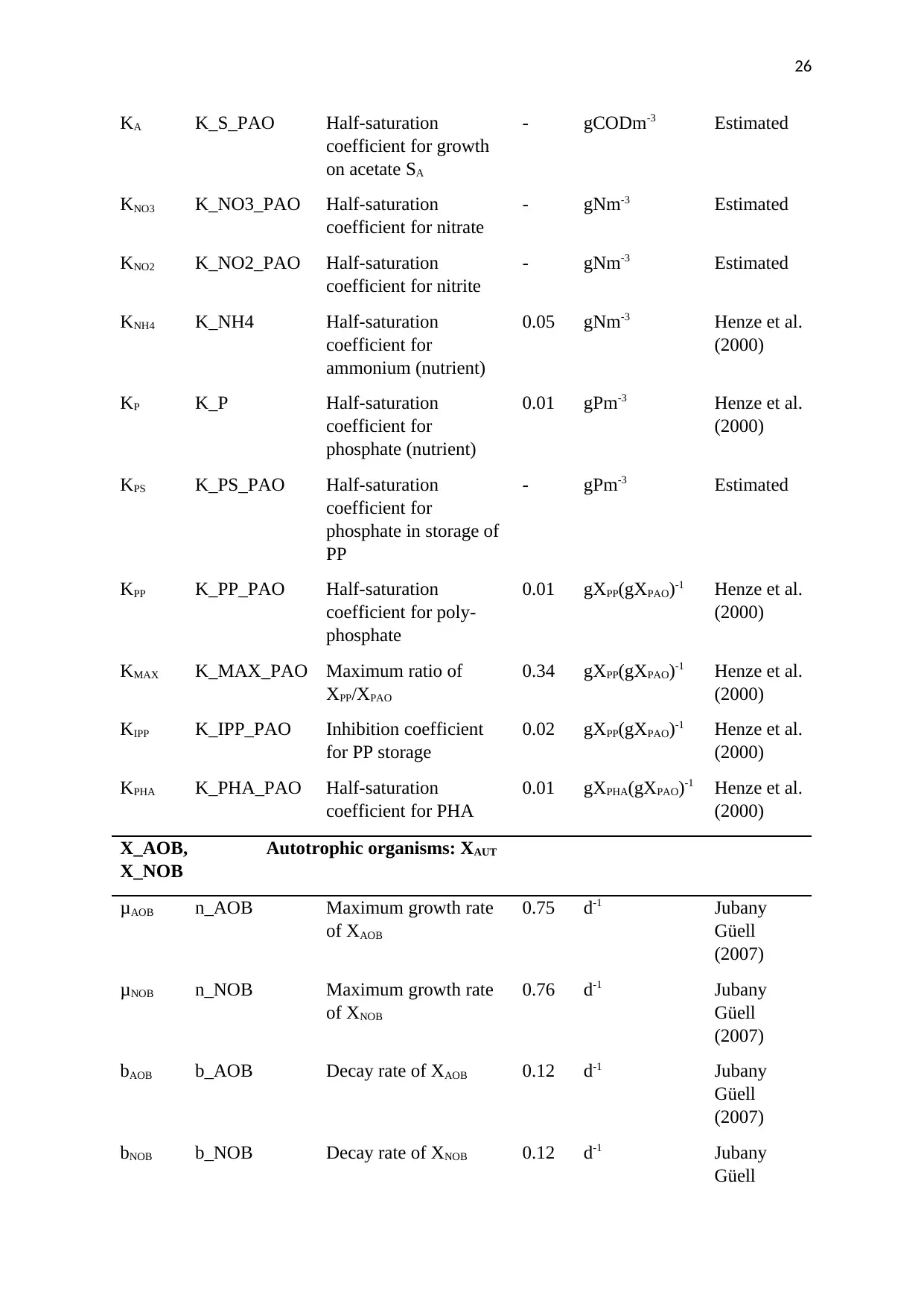
KA K_S_PAO Half-saturation
coefficient for growth
on acetate SA
- gCODm-3 Estimated
KNO3 K_NO3_PAO Half-saturation
coefficient for nitrate
- gNm-3 Estimated
KNO2 K_NO2_PAO Half-saturation
coefficient for nitrite
- gNm-3 Estimated
KNH4 K_NH4 Half-saturation
coefficient for
ammonium (nutrient)
0.05 gNm-3 Henze et al.
(2000)
KP K_P Half-saturation
coefficient for
phosphate (nutrient)
0.01 gPm-3 Henze et al.
(2000)
KPS K_PS_PAO Half-saturation
coefficient for
phosphate in storage of
PP
- gPm-3 Estimated
KPP K_PP_PAO Half-saturation
coefficient for poly-
phosphate
0.01 gXPP(gXPAO)-1 Henze et al.
(2000)
KMAX K_MAX_PAO Maximum ratio of
XPP/XPAO
0.34 gXPP(gXPAO)-1 Henze et al.
(2000)
KIPP K_IPP_PAO Inhibition coefficient
for PP storage
0.02 gXPP(gXPAO)-1 Henze et al.
(2000)
KPHA K_PHA_PAO Half-saturation
coefficient for PHA
0.01 gXPHA(gXPAO)-1 Henze et al.
(2000)
X_AOB,
X_NOB
Autotrophic organisms: XAUT
μAOB n_AOB Maximum growth rate
of XAOB
0.75 d-1 Jubany
Güell
(2007)
μNOB n_NOB Maximum growth rate
of XNOB
0.76 d-1 Jubany
Güell
(2007)
bAOB b_AOB Decay rate of XAOB 0.12 d-1 Jubany
Güell
(2007)
bNOB b_NOB Decay rate of XNOB 0.12 d-1 Jubany
Güell
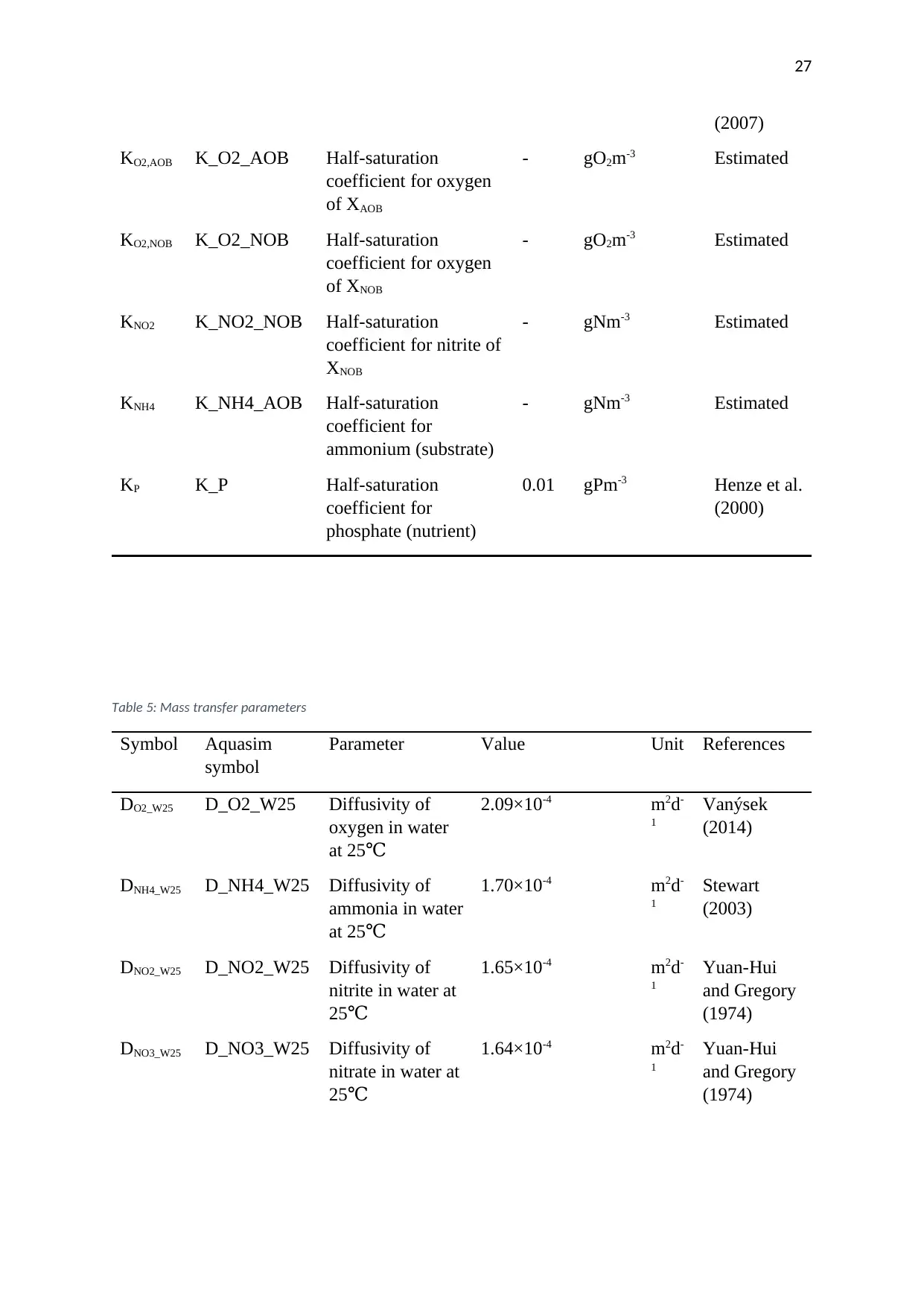
(2007)
KO2,AOB K_O2_AOB Half-saturation
coefficient for oxygen
of XAOB
- gO2m-3 Estimated
KO2,NOB K_O2_NOB Half-saturation
coefficient for oxygen
of XNOB
- gO2m-3 Estimated
KNO2 K_NO2_NOB Half-saturation
coefficient for nitrite of
XNOB
- gNm-3 Estimated
KNH4 K_NH4_AOB Half-saturation
coefficient for
ammonium (substrate)
- gNm-3 Estimated
KP K_P Half-saturation
coefficient for
phosphate (nutrient)
0.01 gPm-3 Henze et al.
(2000)
Table 5: Mass transfer parameters
Symbol Aquasim
symbol
Parameter Value Unit References
DO2_W25 D_O2_W25 Diffusivity of
oxygen in water
at 25℃
2.09×10-4 m2d-
1
Vanýsek
(2014)
DNH4_W25 D_NH4_W25 Diffusivity of
ammonia in water
at 25℃
1.70×10-4 m2d-
1
Stewart
(2003)
DNO2_W25 D_NO2_W25 Diffusivity of
nitrite in water at
25℃
1.65×10-4 m2d-
1
Yuan-Hui
and Gregory
(1974)
DNO3_W25 D_NO3_W25 Diffusivity of
nitrate in water at
25℃
1.64×10-4 m2d-
1
Yuan-Hui
and Gregory
(1974)
⊘ This is a preview!⊘
Do you want full access?
Subscribe today to unlock all pages.

Trusted by 1+ million students worldwide
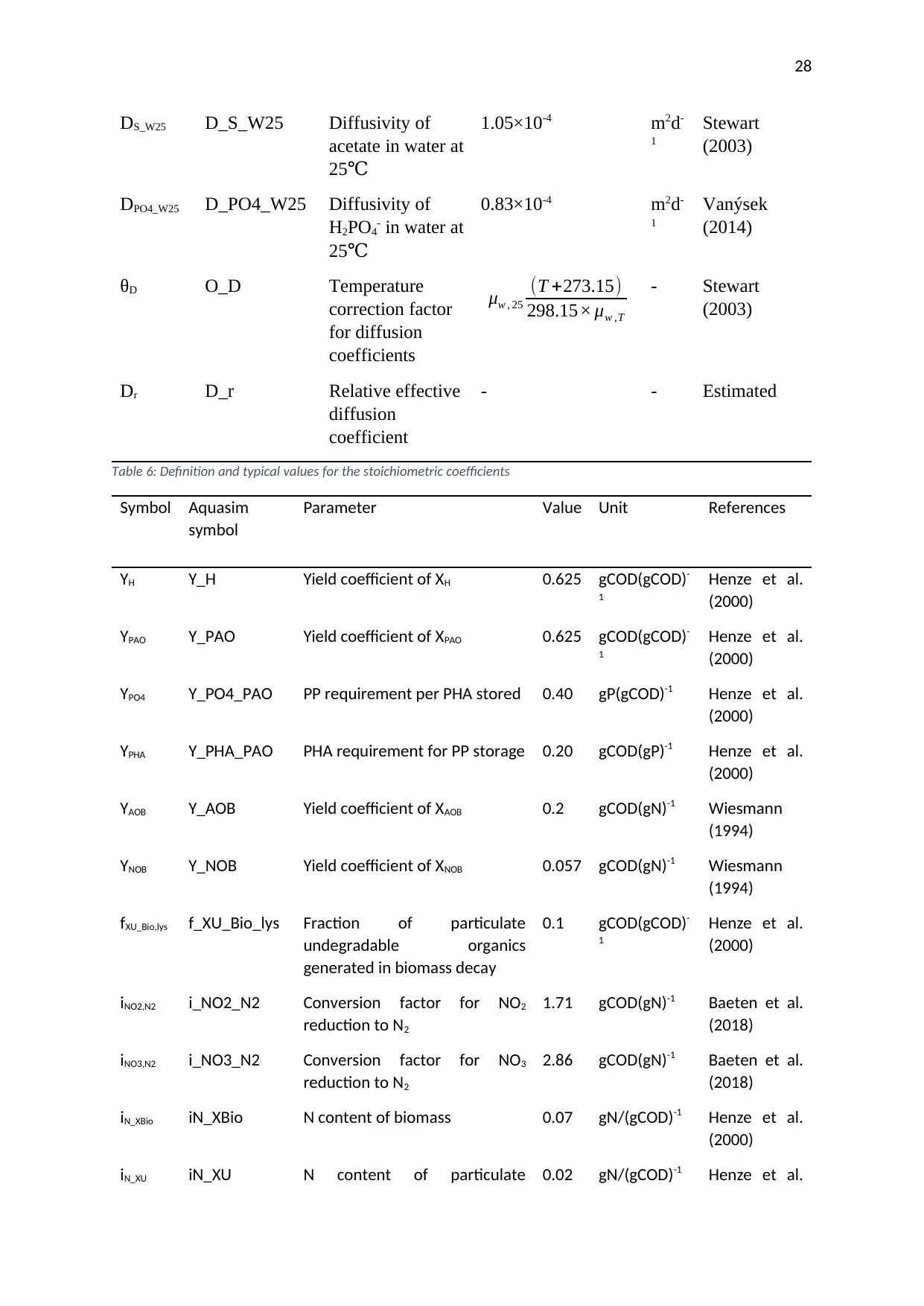
DS_W25 D_S_W25 Diffusivity of
acetate in water at
25℃
1.05×10-4 m2d-
1
Stewart
(2003)
DPO4_W25 D_PO4_W25 Diffusivity of
H2PO4- in water at
25℃
0.83×10-4 m2d-
1
Vanýsek
(2014)
θD O_D Temperature
correction factor
for diffusion
coefficients
μw , 25
(T +273.15)
298.15× μw ,T
- Stewart
(2003)
Dr D_r Relative effective
diffusion
coefficient
- - Estimated
Table 6: Definition and typical values for the stoichiometric coefficients
Symbol Aquasim
symbol
Parameter Value Unit References
YH Y_H Yield coefficient of XH 0.625 gCOD(gCOD)-
1
Henze et al.
(2000)
YPAO Y_PAO Yield coefficient of XPAO 0.625 gCOD(gCOD)-
1
Henze et al.
(2000)
YPO4 Y_PO4_PAO PP requirement per PHA stored 0.40 gP(gCOD)-1 Henze et al.
(2000)
YPHA Y_PHA_PAO PHA requirement for PP storage 0.20 gCOD(gP)-1 Henze et al.
(2000)
YAOB Y_AOB Yield coefficient of XAOB 0.2 gCOD(gN)-1 Wiesmann
(1994)
YNOB Y_NOB Yield coefficient of XNOB 0.057 gCOD(gN)-1 Wiesmann
(1994)
fXU_Bio,lys f_XU_Bio_lys Fraction of particulate
undegradable organics
generated in biomass decay
0.1 gCOD(gCOD)-
1
Henze et al.
(2000)
iNO2,N2 i_NO2_N2 Conversion factor for NO2
reduction to N2
1.71 gCOD(gN)-1 Baeten et al.
(2018)
iNO3,N2 i_NO3_N2 Conversion factor for NO3
reduction to N2
2.86 gCOD(gN)-1 Baeten et al.
(2018)
iN_XBio iN_XBio N content of biomass 0.07 gN/(gCOD)-1 Henze et al.
(2000)
iN_XU iN_XU N content of particulate 0.02 gN/(gCOD)-1 Henze et al.
Paraphrase This Document
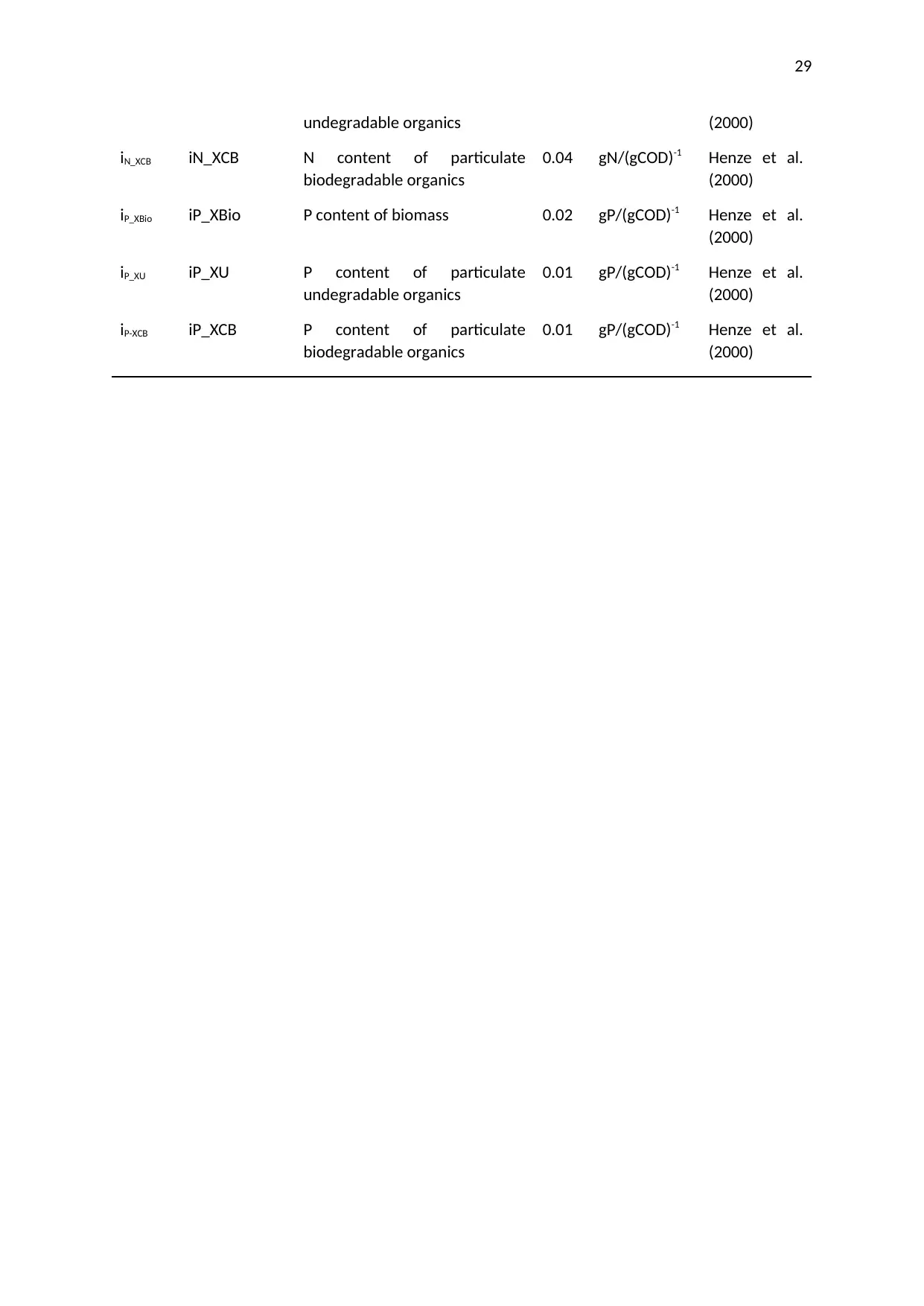
undegradable organics (2000)
iN_XCB iN_XCB N content of particulate
biodegradable organics
0.04 gN/(gCOD)-1 Henze et al.
(2000)
iP_XBio iP_XBio P content of biomass 0.02 gP/(gCOD)-1 Henze et al.
(2000)
iP_XU iP_XU P content of particulate
undegradable organics
0.01 gP/(gCOD)-1 Henze et al.
(2000)
iP-XCB iP_XCB P content of particulate
biodegradable organics
0.01 gP/(gCOD)-1 Henze et al.
(2000)

Your All-in-One AI-Powered Toolkit for Academic Success.
+13062052269
info@desklib.com
Available 24*7 on WhatsApp / Email
© 2024 | Zucol Services PVT LTD | All rights reserved.