Blood Transfusion and Storage Lesions
VerifiedAdded on 2020/05/16
|85
|26859
|109
AI Summary
This assignment delves into the complexities of blood transfusion, particularly focusing on the detrimental effects that blood storage can have on red blood cell function. It examines alterations in glycolysis, membrane structure, and antioxidant capacity during storage. The impact of these changes on microvascular response and overall clinical outcomes is also explored.
Contribute Materials
Your contribution can guide someone’s learning journey. Share your
documents today.
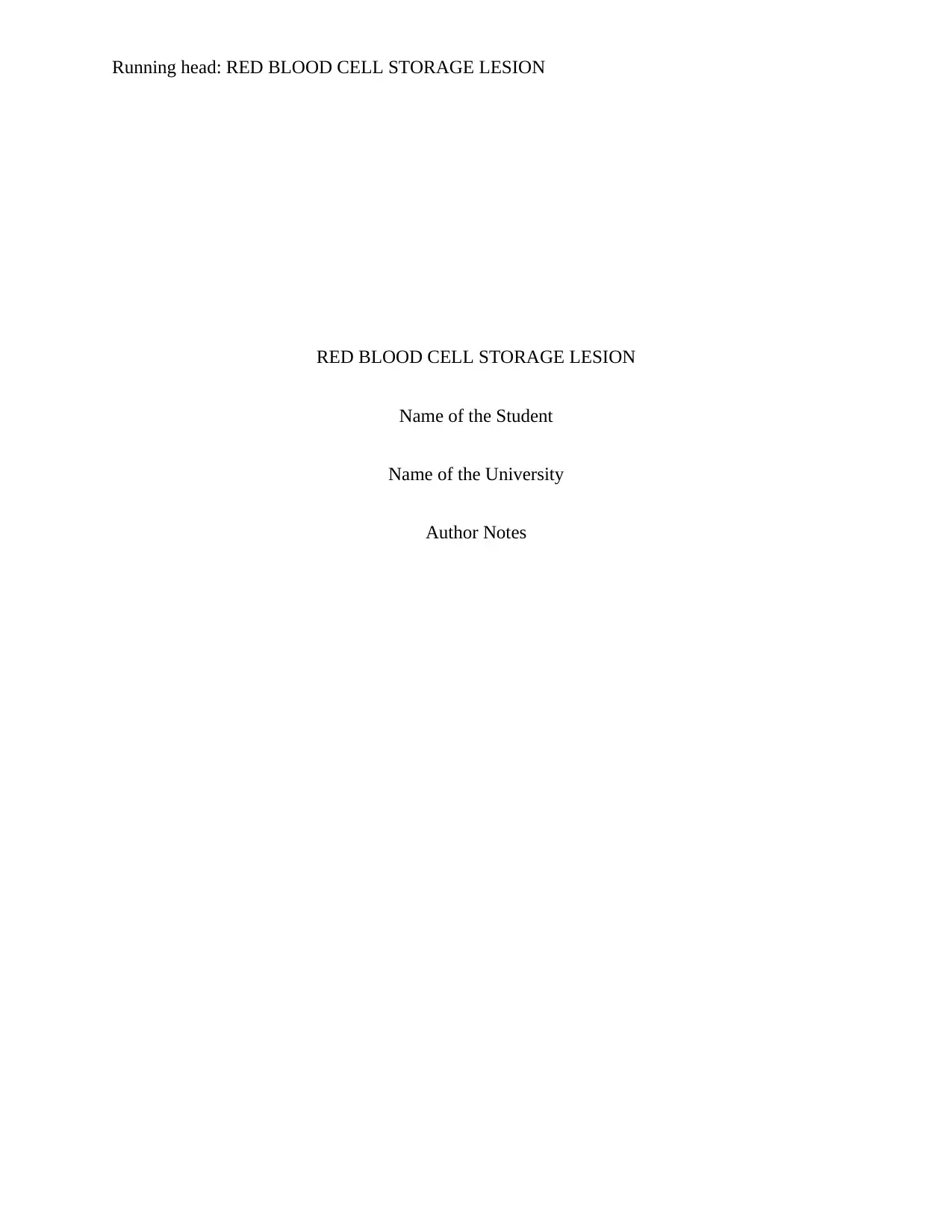
Running head: RED BLOOD CELL STORAGE LESION
RED BLOOD CELL STORAGE LESION
Name of the Student
Name of the University
Author Notes
RED BLOOD CELL STORAGE LESION
Name of the Student
Name of the University
Author Notes
Secure Best Marks with AI Grader
Need help grading? Try our AI Grader for instant feedback on your assignments.
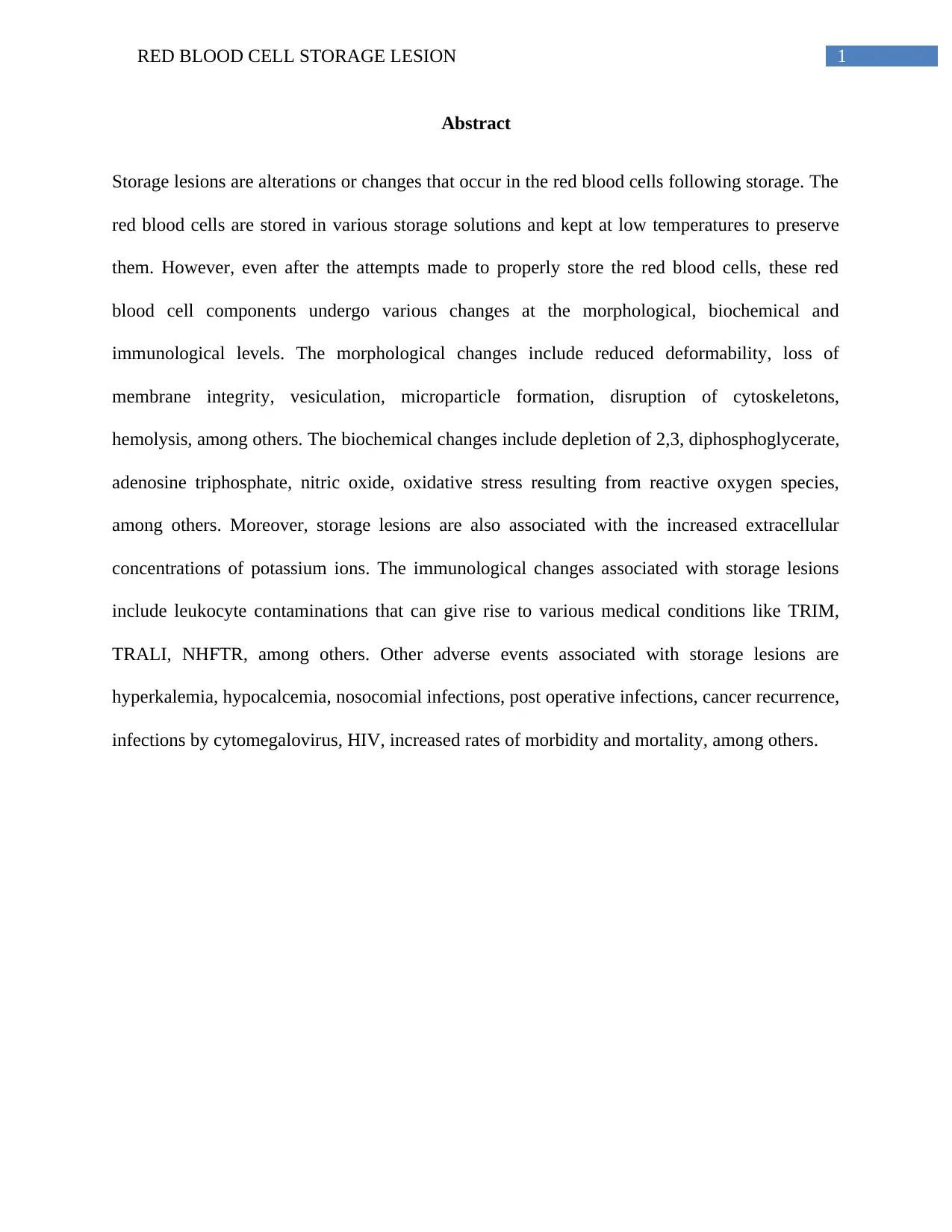
1RED BLOOD CELL STORAGE LESION
Abstract
Storage lesions are alterations or changes that occur in the red blood cells following storage. The
red blood cells are stored in various storage solutions and kept at low temperatures to preserve
them. However, even after the attempts made to properly store the red blood cells, these red
blood cell components undergo various changes at the morphological, biochemical and
immunological levels. The morphological changes include reduced deformability, loss of
membrane integrity, vesiculation, microparticle formation, disruption of cytoskeletons,
hemolysis, among others. The biochemical changes include depletion of 2,3, diphosphoglycerate,
adenosine triphosphate, nitric oxide, oxidative stress resulting from reactive oxygen species,
among others. Moreover, storage lesions are also associated with the increased extracellular
concentrations of potassium ions. The immunological changes associated with storage lesions
include leukocyte contaminations that can give rise to various medical conditions like TRIM,
TRALI, NHFTR, among others. Other adverse events associated with storage lesions are
hyperkalemia, hypocalcemia, nosocomial infections, post operative infections, cancer recurrence,
infections by cytomegalovirus, HIV, increased rates of morbidity and mortality, among others.
Abstract
Storage lesions are alterations or changes that occur in the red blood cells following storage. The
red blood cells are stored in various storage solutions and kept at low temperatures to preserve
them. However, even after the attempts made to properly store the red blood cells, these red
blood cell components undergo various changes at the morphological, biochemical and
immunological levels. The morphological changes include reduced deformability, loss of
membrane integrity, vesiculation, microparticle formation, disruption of cytoskeletons,
hemolysis, among others. The biochemical changes include depletion of 2,3, diphosphoglycerate,
adenosine triphosphate, nitric oxide, oxidative stress resulting from reactive oxygen species,
among others. Moreover, storage lesions are also associated with the increased extracellular
concentrations of potassium ions. The immunological changes associated with storage lesions
include leukocyte contaminations that can give rise to various medical conditions like TRIM,
TRALI, NHFTR, among others. Other adverse events associated with storage lesions are
hyperkalemia, hypocalcemia, nosocomial infections, post operative infections, cancer recurrence,
infections by cytomegalovirus, HIV, increased rates of morbidity and mortality, among others.
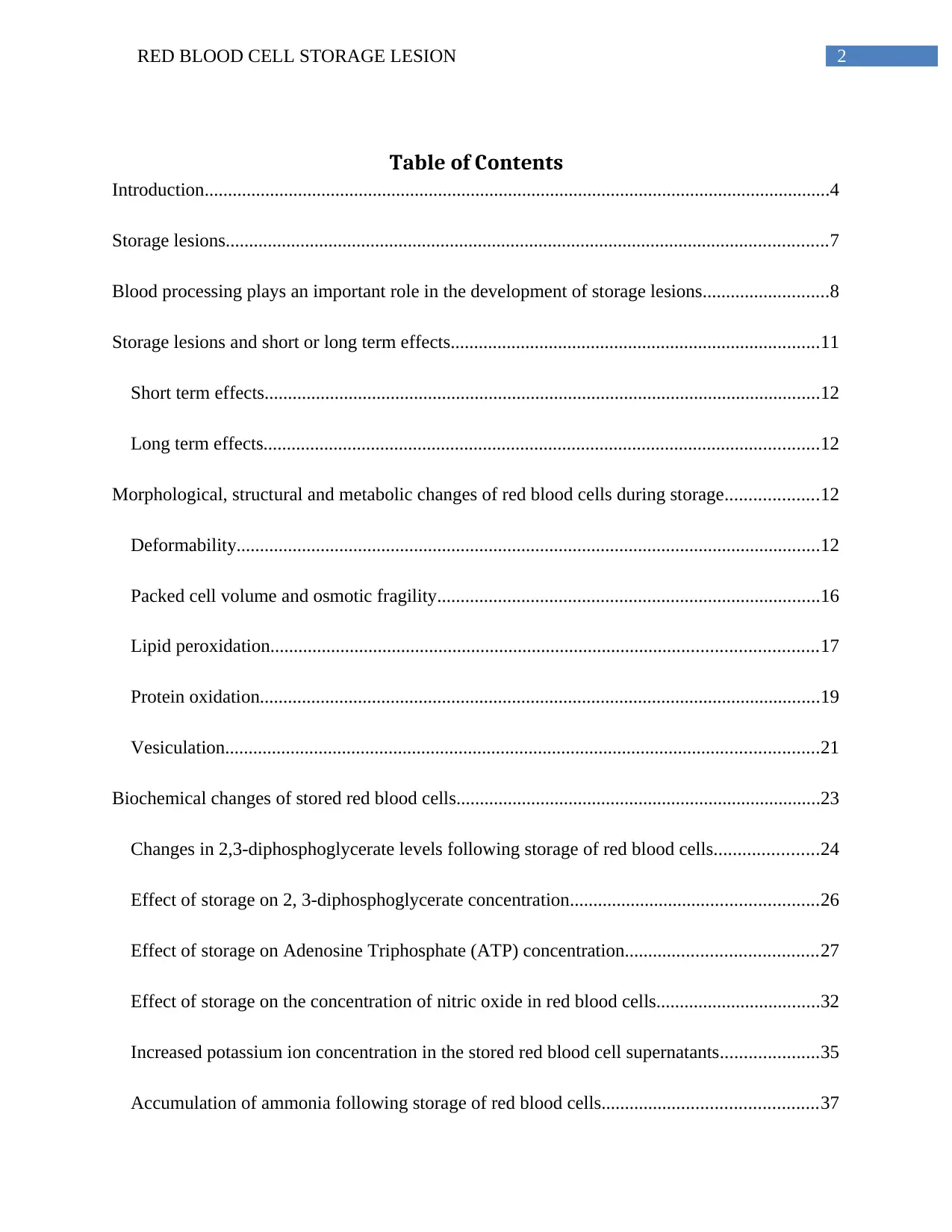
2RED BLOOD CELL STORAGE LESION
Table of Contents
Introduction......................................................................................................................................4
Storage lesions.................................................................................................................................7
Blood processing plays an important role in the development of storage lesions...........................8
Storage lesions and short or long term effects...............................................................................11
Short term effects.......................................................................................................................12
Long term effects.......................................................................................................................12
Morphological, structural and metabolic changes of red blood cells during storage....................12
Deformability.............................................................................................................................12
Packed cell volume and osmotic fragility..................................................................................16
Lipid peroxidation.....................................................................................................................17
Protein oxidation........................................................................................................................19
Vesiculation...............................................................................................................................21
Biochemical changes of stored red blood cells..............................................................................23
Changes in 2,3-diphosphoglycerate levels following storage of red blood cells......................24
Effect of storage on 2, 3-diphosphoglycerate concentration.....................................................26
Effect of storage on Adenosine Triphosphate (ATP) concentration.........................................27
Effect of storage on the concentration of nitric oxide in red blood cells...................................32
Increased potassium ion concentration in the stored red blood cell supernatants.....................35
Accumulation of ammonia following storage of red blood cells..............................................37
Table of Contents
Introduction......................................................................................................................................4
Storage lesions.................................................................................................................................7
Blood processing plays an important role in the development of storage lesions...........................8
Storage lesions and short or long term effects...............................................................................11
Short term effects.......................................................................................................................12
Long term effects.......................................................................................................................12
Morphological, structural and metabolic changes of red blood cells during storage....................12
Deformability.............................................................................................................................12
Packed cell volume and osmotic fragility..................................................................................16
Lipid peroxidation.....................................................................................................................17
Protein oxidation........................................................................................................................19
Vesiculation...............................................................................................................................21
Biochemical changes of stored red blood cells..............................................................................23
Changes in 2,3-diphosphoglycerate levels following storage of red blood cells......................24
Effect of storage on 2, 3-diphosphoglycerate concentration.....................................................26
Effect of storage on Adenosine Triphosphate (ATP) concentration.........................................27
Effect of storage on the concentration of nitric oxide in red blood cells...................................32
Increased potassium ion concentration in the stored red blood cell supernatants.....................35
Accumulation of ammonia following storage of red blood cells..............................................37
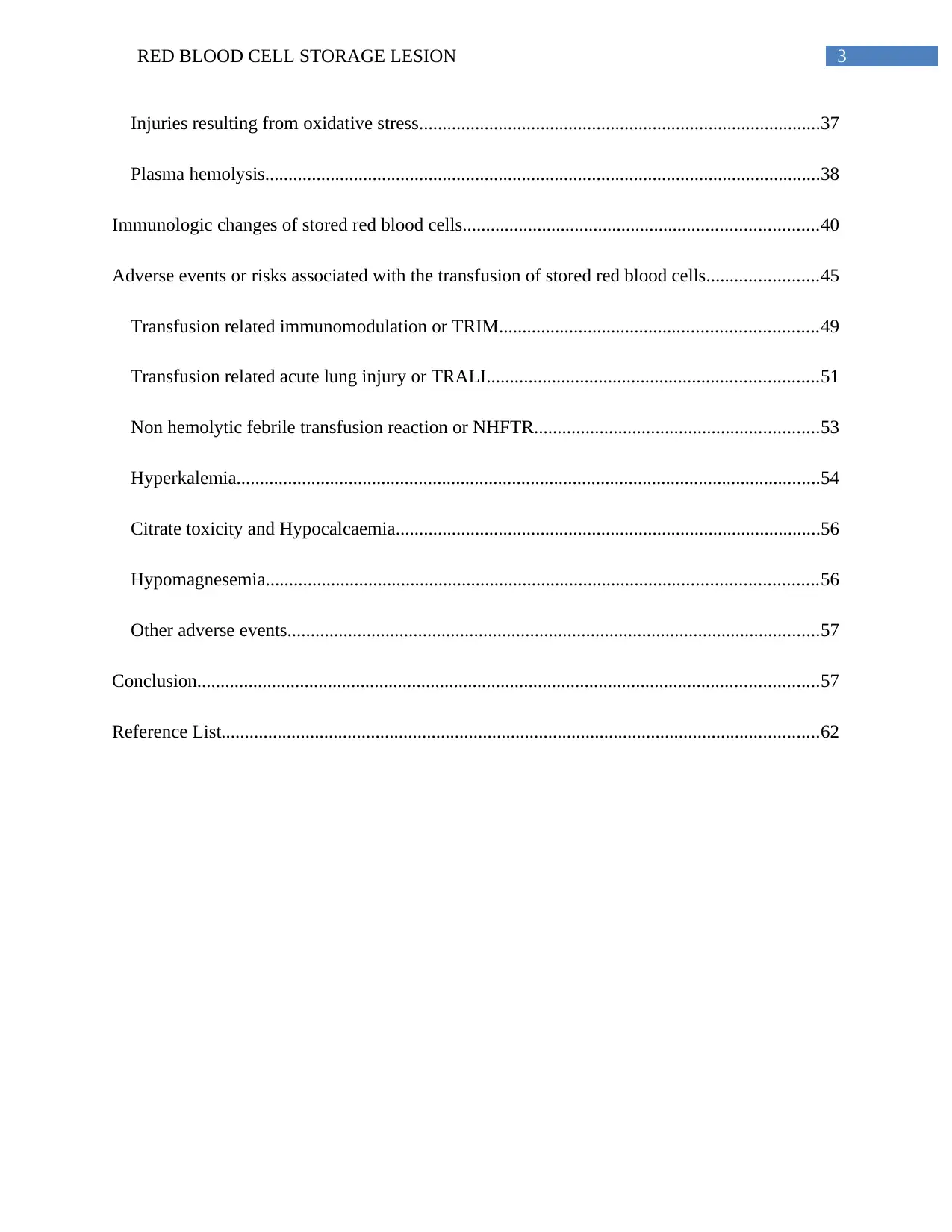
3RED BLOOD CELL STORAGE LESION
Injuries resulting from oxidative stress......................................................................................37
Plasma hemolysis.......................................................................................................................38
Immunologic changes of stored red blood cells............................................................................40
Adverse events or risks associated with the transfusion of stored red blood cells........................45
Transfusion related immunomodulation or TRIM....................................................................49
Transfusion related acute lung injury or TRALI.......................................................................51
Non hemolytic febrile transfusion reaction or NHFTR.............................................................53
Hyperkalemia.............................................................................................................................54
Citrate toxicity and Hypocalcaemia...........................................................................................56
Hypomagnesemia......................................................................................................................56
Other adverse events..................................................................................................................57
Conclusion.....................................................................................................................................57
Reference List................................................................................................................................62
Injuries resulting from oxidative stress......................................................................................37
Plasma hemolysis.......................................................................................................................38
Immunologic changes of stored red blood cells............................................................................40
Adverse events or risks associated with the transfusion of stored red blood cells........................45
Transfusion related immunomodulation or TRIM....................................................................49
Transfusion related acute lung injury or TRALI.......................................................................51
Non hemolytic febrile transfusion reaction or NHFTR.............................................................53
Hyperkalemia.............................................................................................................................54
Citrate toxicity and Hypocalcaemia...........................................................................................56
Hypomagnesemia......................................................................................................................56
Other adverse events..................................................................................................................57
Conclusion.....................................................................................................................................57
Reference List................................................................................................................................62
Secure Best Marks with AI Grader
Need help grading? Try our AI Grader for instant feedback on your assignments.
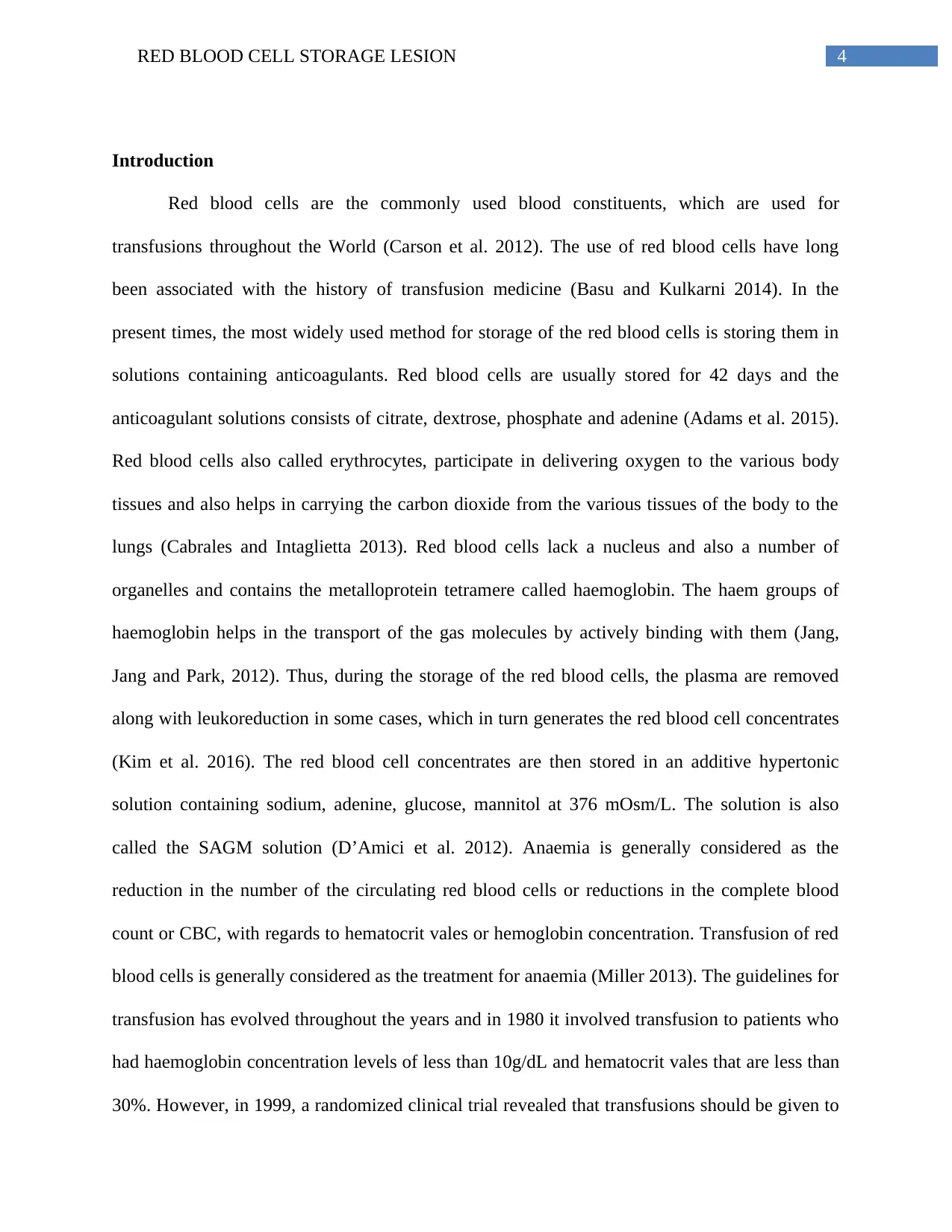
4RED BLOOD CELL STORAGE LESION
Introduction
Red blood cells are the commonly used blood constituents, which are used for
transfusions throughout the World (Carson et al. 2012). The use of red blood cells have long
been associated with the history of transfusion medicine (Basu and Kulkarni 2014). In the
present times, the most widely used method for storage of the red blood cells is storing them in
solutions containing anticoagulants. Red blood cells are usually stored for 42 days and the
anticoagulant solutions consists of citrate, dextrose, phosphate and adenine (Adams et al. 2015).
Red blood cells also called erythrocytes, participate in delivering oxygen to the various body
tissues and also helps in carrying the carbon dioxide from the various tissues of the body to the
lungs (Cabrales and Intaglietta 2013). Red blood cells lack a nucleus and also a number of
organelles and contains the metalloprotein tetramere called haemoglobin. The haem groups of
haemoglobin helps in the transport of the gas molecules by actively binding with them (Jang,
Jang and Park, 2012). Thus, during the storage of the red blood cells, the plasma are removed
along with leukoreduction in some cases, which in turn generates the red blood cell concentrates
(Kim et al. 2016). The red blood cell concentrates are then stored in an additive hypertonic
solution containing sodium, adenine, glucose, mannitol at 376 mOsm/L. The solution is also
called the SAGM solution (D’Amici et al. 2012). Anaemia is generally considered as the
reduction in the number of the circulating red blood cells or reductions in the complete blood
count or CBC, with regards to hematocrit vales or hemoglobin concentration. Transfusion of red
blood cells is generally considered as the treatment for anaemia (Miller 2013). The guidelines for
transfusion has evolved throughout the years and in 1980 it involved transfusion to patients who
had haemoglobin concentration levels of less than 10g/dL and hematocrit vales that are less than
30%. However, in 1999, a randomized clinical trial revealed that transfusions should be given to
Introduction
Red blood cells are the commonly used blood constituents, which are used for
transfusions throughout the World (Carson et al. 2012). The use of red blood cells have long
been associated with the history of transfusion medicine (Basu and Kulkarni 2014). In the
present times, the most widely used method for storage of the red blood cells is storing them in
solutions containing anticoagulants. Red blood cells are usually stored for 42 days and the
anticoagulant solutions consists of citrate, dextrose, phosphate and adenine (Adams et al. 2015).
Red blood cells also called erythrocytes, participate in delivering oxygen to the various body
tissues and also helps in carrying the carbon dioxide from the various tissues of the body to the
lungs (Cabrales and Intaglietta 2013). Red blood cells lack a nucleus and also a number of
organelles and contains the metalloprotein tetramere called haemoglobin. The haem groups of
haemoglobin helps in the transport of the gas molecules by actively binding with them (Jang,
Jang and Park, 2012). Thus, during the storage of the red blood cells, the plasma are removed
along with leukoreduction in some cases, which in turn generates the red blood cell concentrates
(Kim et al. 2016). The red blood cell concentrates are then stored in an additive hypertonic
solution containing sodium, adenine, glucose, mannitol at 376 mOsm/L. The solution is also
called the SAGM solution (D’Amici et al. 2012). Anaemia is generally considered as the
reduction in the number of the circulating red blood cells or reductions in the complete blood
count or CBC, with regards to hematocrit vales or hemoglobin concentration. Transfusion of red
blood cells is generally considered as the treatment for anaemia (Miller 2013). The guidelines for
transfusion has evolved throughout the years and in 1980 it involved transfusion to patients who
had haemoglobin concentration levels of less than 10g/dL and hematocrit vales that are less than
30%. However, in 1999, a randomized clinical trial revealed that transfusions should be given to
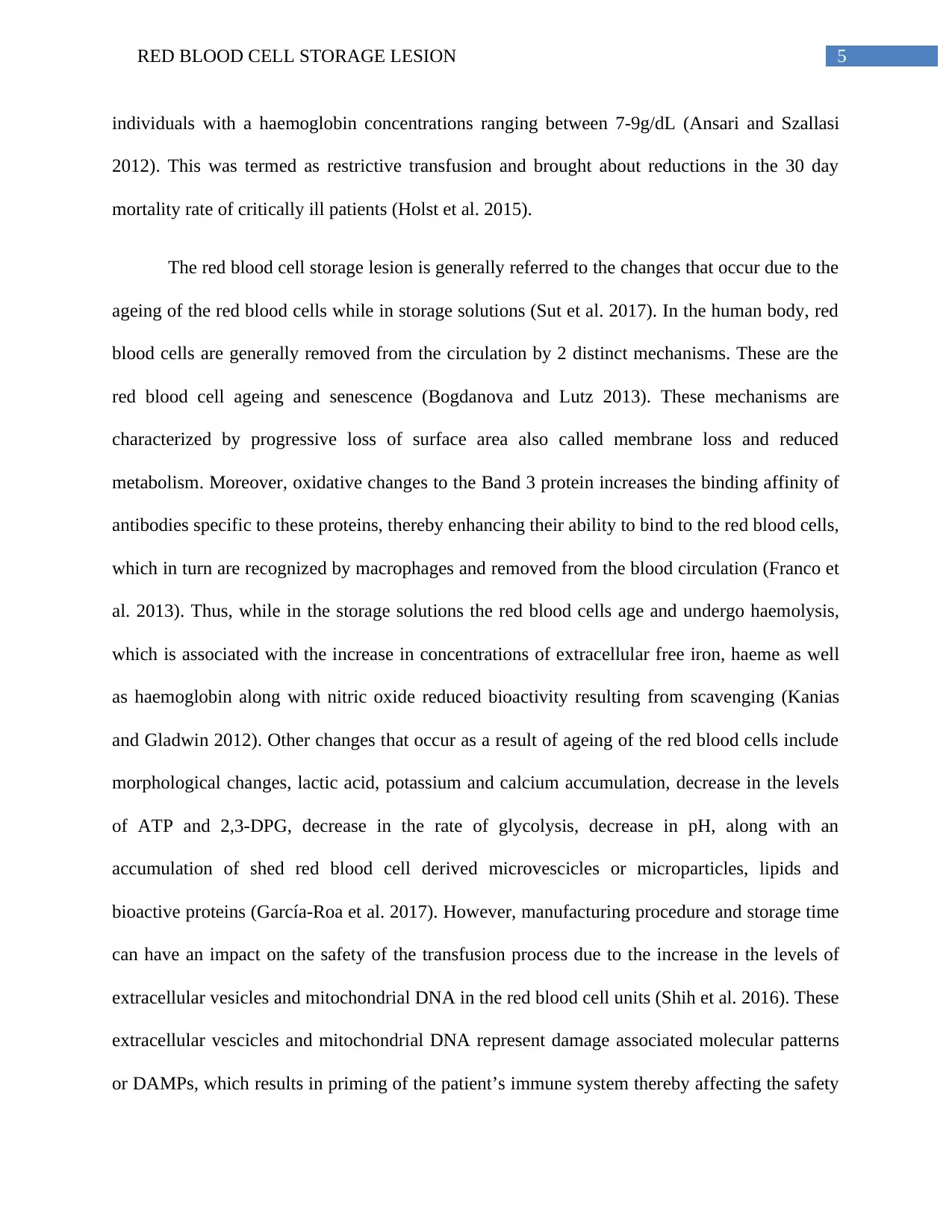
5RED BLOOD CELL STORAGE LESION
individuals with a haemoglobin concentrations ranging between 7-9g/dL (Ansari and Szallasi
2012). This was termed as restrictive transfusion and brought about reductions in the 30 day
mortality rate of critically ill patients (Holst et al. 2015).
The red blood cell storage lesion is generally referred to the changes that occur due to the
ageing of the red blood cells while in storage solutions (Sut et al. 2017). In the human body, red
blood cells are generally removed from the circulation by 2 distinct mechanisms. These are the
red blood cell ageing and senescence (Bogdanova and Lutz 2013). These mechanisms are
characterized by progressive loss of surface area also called membrane loss and reduced
metabolism. Moreover, oxidative changes to the Band 3 protein increases the binding affinity of
antibodies specific to these proteins, thereby enhancing their ability to bind to the red blood cells,
which in turn are recognized by macrophages and removed from the blood circulation (Franco et
al. 2013). Thus, while in the storage solutions the red blood cells age and undergo haemolysis,
which is associated with the increase in concentrations of extracellular free iron, haeme as well
as haemoglobin along with nitric oxide reduced bioactivity resulting from scavenging (Kanias
and Gladwin 2012). Other changes that occur as a result of ageing of the red blood cells include
morphological changes, lactic acid, potassium and calcium accumulation, decrease in the levels
of ATP and 2,3-DPG, decrease in the rate of glycolysis, decrease in pH, along with an
accumulation of shed red blood cell derived microvescicles or microparticles, lipids and
bioactive proteins (García-Roa et al. 2017). However, manufacturing procedure and storage time
can have an impact on the safety of the transfusion process due to the increase in the levels of
extracellular vesicles and mitochondrial DNA in the red blood cell units (Shih et al. 2016). These
extracellular vescicles and mitochondrial DNA represent damage associated molecular patterns
or DAMPs, which results in priming of the patient’s immune system thereby affecting the safety
individuals with a haemoglobin concentrations ranging between 7-9g/dL (Ansari and Szallasi
2012). This was termed as restrictive transfusion and brought about reductions in the 30 day
mortality rate of critically ill patients (Holst et al. 2015).
The red blood cell storage lesion is generally referred to the changes that occur due to the
ageing of the red blood cells while in storage solutions (Sut et al. 2017). In the human body, red
blood cells are generally removed from the circulation by 2 distinct mechanisms. These are the
red blood cell ageing and senescence (Bogdanova and Lutz 2013). These mechanisms are
characterized by progressive loss of surface area also called membrane loss and reduced
metabolism. Moreover, oxidative changes to the Band 3 protein increases the binding affinity of
antibodies specific to these proteins, thereby enhancing their ability to bind to the red blood cells,
which in turn are recognized by macrophages and removed from the blood circulation (Franco et
al. 2013). Thus, while in the storage solutions the red blood cells age and undergo haemolysis,
which is associated with the increase in concentrations of extracellular free iron, haeme as well
as haemoglobin along with nitric oxide reduced bioactivity resulting from scavenging (Kanias
and Gladwin 2012). Other changes that occur as a result of ageing of the red blood cells include
morphological changes, lactic acid, potassium and calcium accumulation, decrease in the levels
of ATP and 2,3-DPG, decrease in the rate of glycolysis, decrease in pH, along with an
accumulation of shed red blood cell derived microvescicles or microparticles, lipids and
bioactive proteins (García-Roa et al. 2017). However, manufacturing procedure and storage time
can have an impact on the safety of the transfusion process due to the increase in the levels of
extracellular vesicles and mitochondrial DNA in the red blood cell units (Shih et al. 2016). These
extracellular vescicles and mitochondrial DNA represent damage associated molecular patterns
or DAMPs, which results in priming of the patient’s immune system thereby affecting the safety
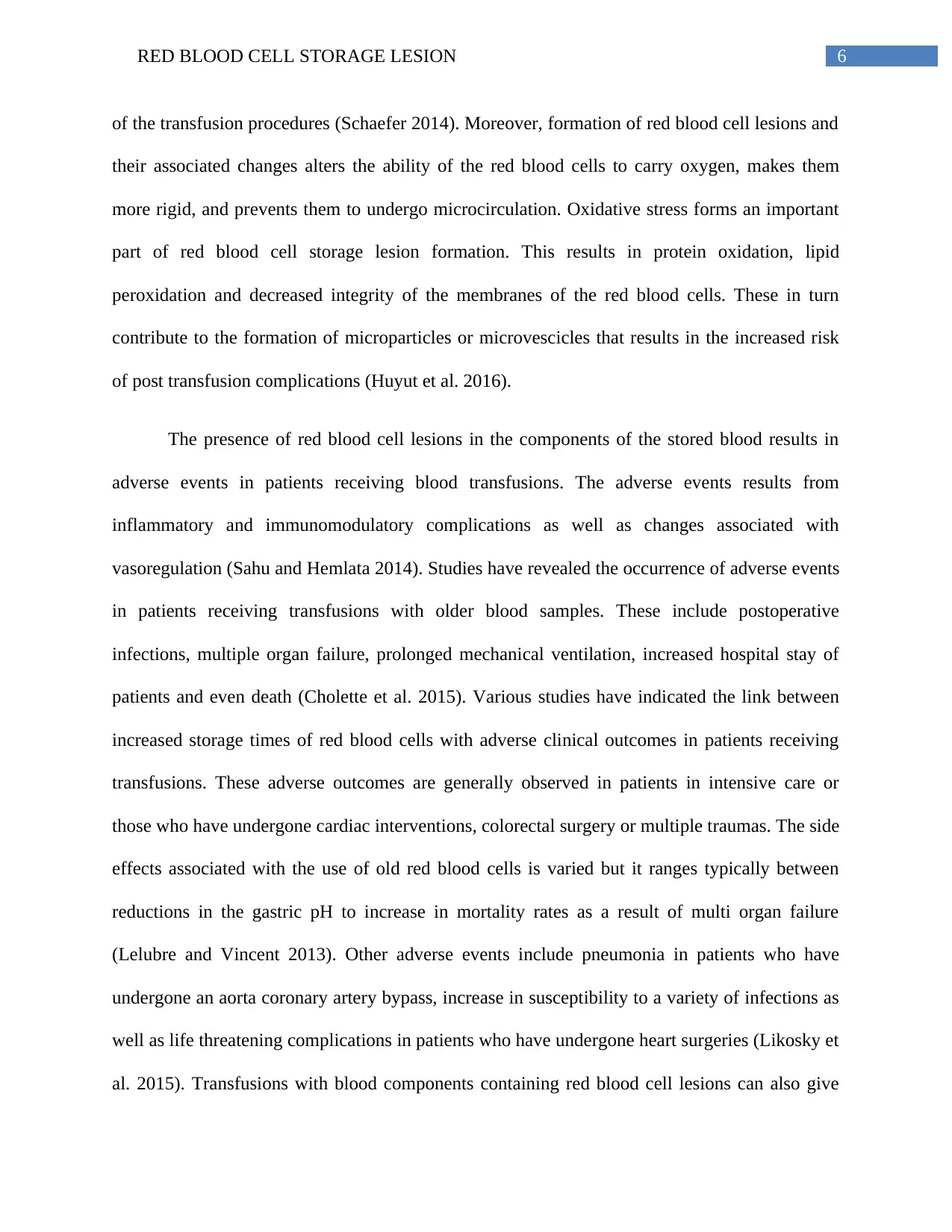
6RED BLOOD CELL STORAGE LESION
of the transfusion procedures (Schaefer 2014). Moreover, formation of red blood cell lesions and
their associated changes alters the ability of the red blood cells to carry oxygen, makes them
more rigid, and prevents them to undergo microcirculation. Oxidative stress forms an important
part of red blood cell storage lesion formation. This results in protein oxidation, lipid
peroxidation and decreased integrity of the membranes of the red blood cells. These in turn
contribute to the formation of microparticles or microvescicles that results in the increased risk
of post transfusion complications (Huyut et al. 2016).
The presence of red blood cell lesions in the components of the stored blood results in
adverse events in patients receiving blood transfusions. The adverse events results from
inflammatory and immunomodulatory complications as well as changes associated with
vasoregulation (Sahu and Hemlata 2014). Studies have revealed the occurrence of adverse events
in patients receiving transfusions with older blood samples. These include postoperative
infections, multiple organ failure, prolonged mechanical ventilation, increased hospital stay of
patients and even death (Cholette et al. 2015). Various studies have indicated the link between
increased storage times of red blood cells with adverse clinical outcomes in patients receiving
transfusions. These adverse outcomes are generally observed in patients in intensive care or
those who have undergone cardiac interventions, colorectal surgery or multiple traumas. The side
effects associated with the use of old red blood cells is varied but it ranges typically between
reductions in the gastric pH to increase in mortality rates as a result of multi organ failure
(Lelubre and Vincent 2013). Other adverse events include pneumonia in patients who have
undergone an aorta coronary artery bypass, increase in susceptibility to a variety of infections as
well as life threatening complications in patients who have undergone heart surgeries (Likosky et
al. 2015). Transfusions with blood components containing red blood cell lesions can also give
of the transfusion procedures (Schaefer 2014). Moreover, formation of red blood cell lesions and
their associated changes alters the ability of the red blood cells to carry oxygen, makes them
more rigid, and prevents them to undergo microcirculation. Oxidative stress forms an important
part of red blood cell storage lesion formation. This results in protein oxidation, lipid
peroxidation and decreased integrity of the membranes of the red blood cells. These in turn
contribute to the formation of microparticles or microvescicles that results in the increased risk
of post transfusion complications (Huyut et al. 2016).
The presence of red blood cell lesions in the components of the stored blood results in
adverse events in patients receiving blood transfusions. The adverse events results from
inflammatory and immunomodulatory complications as well as changes associated with
vasoregulation (Sahu and Hemlata 2014). Studies have revealed the occurrence of adverse events
in patients receiving transfusions with older blood samples. These include postoperative
infections, multiple organ failure, prolonged mechanical ventilation, increased hospital stay of
patients and even death (Cholette et al. 2015). Various studies have indicated the link between
increased storage times of red blood cells with adverse clinical outcomes in patients receiving
transfusions. These adverse outcomes are generally observed in patients in intensive care or
those who have undergone cardiac interventions, colorectal surgery or multiple traumas. The side
effects associated with the use of old red blood cells is varied but it ranges typically between
reductions in the gastric pH to increase in mortality rates as a result of multi organ failure
(Lelubre and Vincent 2013). Other adverse events include pneumonia in patients who have
undergone an aorta coronary artery bypass, increase in susceptibility to a variety of infections as
well as life threatening complications in patients who have undergone heart surgeries (Likosky et
al. 2015). Transfusions with blood components containing red blood cell lesions can also give
Paraphrase This Document
Need a fresh take? Get an instant paraphrase of this document with our AI Paraphraser
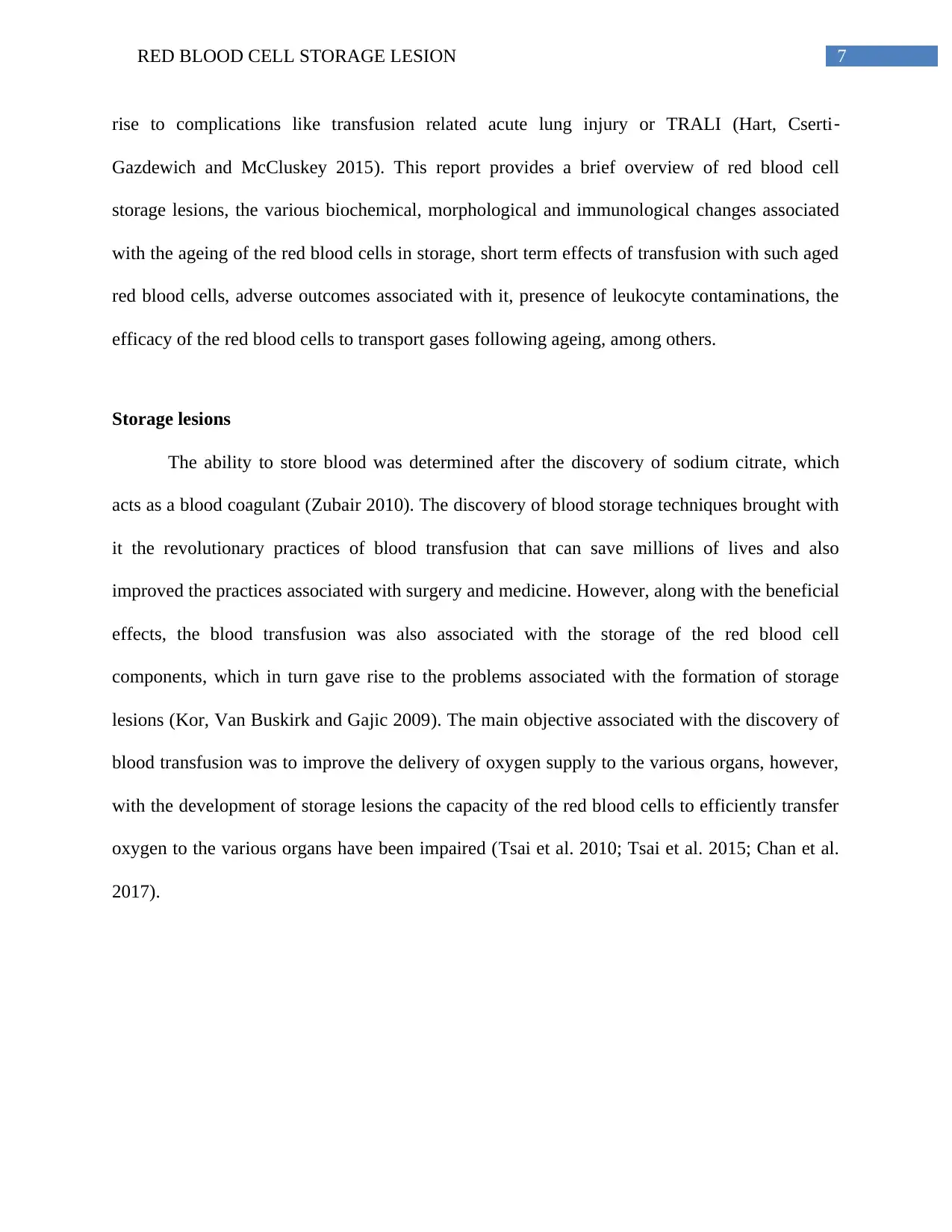
7RED BLOOD CELL STORAGE LESION
rise to complications like transfusion related acute lung injury or TRALI (Hart, Cserti‐
Gazdewich and McCluskey 2015). This report provides a brief overview of red blood cell
storage lesions, the various biochemical, morphological and immunological changes associated
with the ageing of the red blood cells in storage, short term effects of transfusion with such aged
red blood cells, adverse outcomes associated with it, presence of leukocyte contaminations, the
efficacy of the red blood cells to transport gases following ageing, among others.
Storage lesions
The ability to store blood was determined after the discovery of sodium citrate, which
acts as a blood coagulant (Zubair 2010). The discovery of blood storage techniques brought with
it the revolutionary practices of blood transfusion that can save millions of lives and also
improved the practices associated with surgery and medicine. However, along with the beneficial
effects, the blood transfusion was also associated with the storage of the red blood cell
components, which in turn gave rise to the problems associated with the formation of storage
lesions (Kor, Van Buskirk and Gajic 2009). The main objective associated with the discovery of
blood transfusion was to improve the delivery of oxygen supply to the various organs, however,
with the development of storage lesions the capacity of the red blood cells to efficiently transfer
oxygen to the various organs have been impaired (Tsai et al. 2010; Tsai et al. 2015; Chan et al.
2017).
rise to complications like transfusion related acute lung injury or TRALI (Hart, Cserti‐
Gazdewich and McCluskey 2015). This report provides a brief overview of red blood cell
storage lesions, the various biochemical, morphological and immunological changes associated
with the ageing of the red blood cells in storage, short term effects of transfusion with such aged
red blood cells, adverse outcomes associated with it, presence of leukocyte contaminations, the
efficacy of the red blood cells to transport gases following ageing, among others.
Storage lesions
The ability to store blood was determined after the discovery of sodium citrate, which
acts as a blood coagulant (Zubair 2010). The discovery of blood storage techniques brought with
it the revolutionary practices of blood transfusion that can save millions of lives and also
improved the practices associated with surgery and medicine. However, along with the beneficial
effects, the blood transfusion was also associated with the storage of the red blood cell
components, which in turn gave rise to the problems associated with the formation of storage
lesions (Kor, Van Buskirk and Gajic 2009). The main objective associated with the discovery of
blood transfusion was to improve the delivery of oxygen supply to the various organs, however,
with the development of storage lesions the capacity of the red blood cells to efficiently transfer
oxygen to the various organs have been impaired (Tsai et al. 2010; Tsai et al. 2015; Chan et al.
2017).
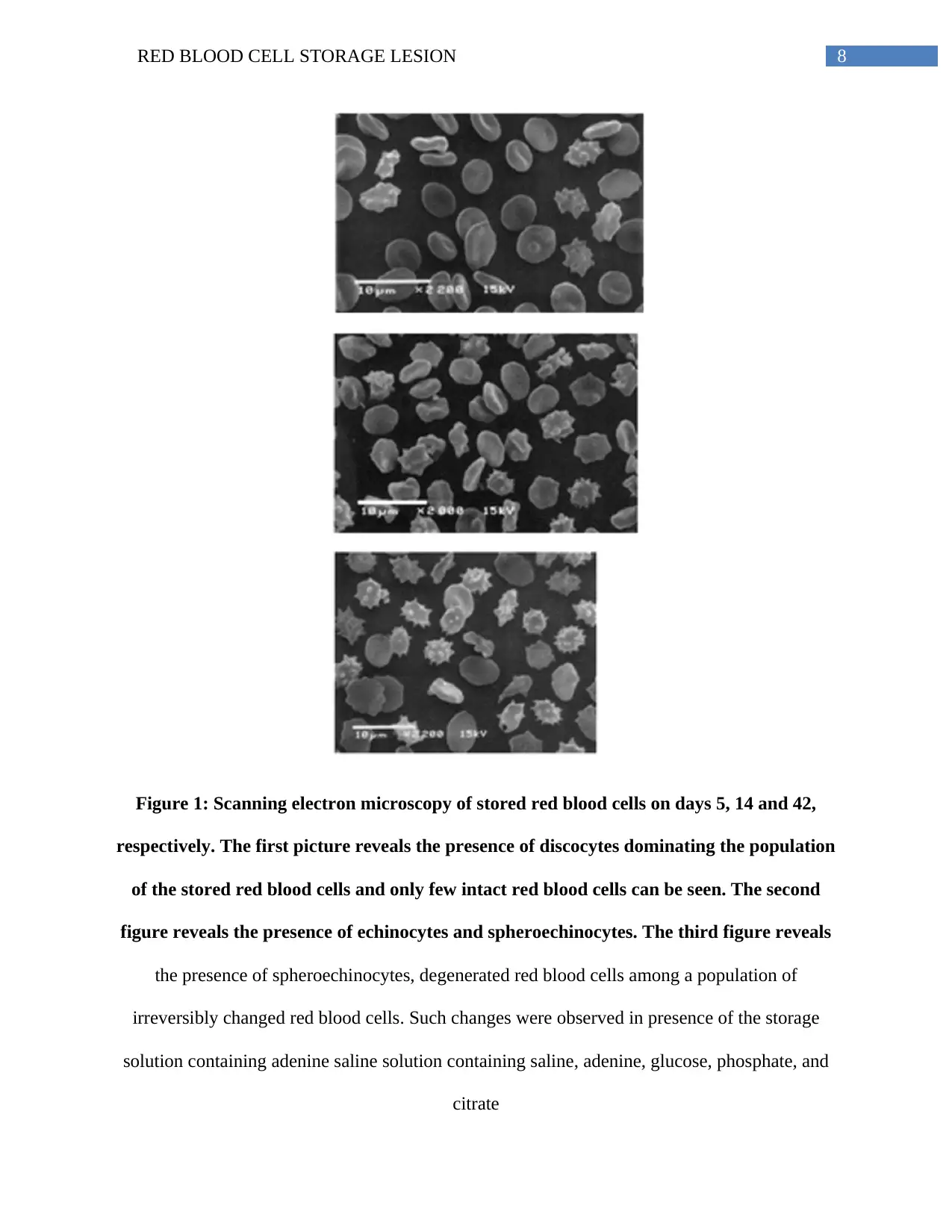
8RED BLOOD CELL STORAGE LESION
Figure 1: Scanning electron microscopy of stored red blood cells on days 5, 14 and 42,
respectively. The first picture reveals the presence of discocytes dominating the population
of the stored red blood cells and only few intact red blood cells can be seen. The second
figure reveals the presence of echinocytes and spheroechinocytes. The third figure reveals
the presence of spheroechinocytes, degenerated red blood cells among a population of
irreversibly changed red blood cells. Such changes were observed in presence of the storage
solution containing adenine saline solution containing saline, adenine, glucose, phosphate, and
citrate
Figure 1: Scanning electron microscopy of stored red blood cells on days 5, 14 and 42,
respectively. The first picture reveals the presence of discocytes dominating the population
of the stored red blood cells and only few intact red blood cells can be seen. The second
figure reveals the presence of echinocytes and spheroechinocytes. The third figure reveals
the presence of spheroechinocytes, degenerated red blood cells among a population of
irreversibly changed red blood cells. Such changes were observed in presence of the storage
solution containing adenine saline solution containing saline, adenine, glucose, phosphate, and
citrate
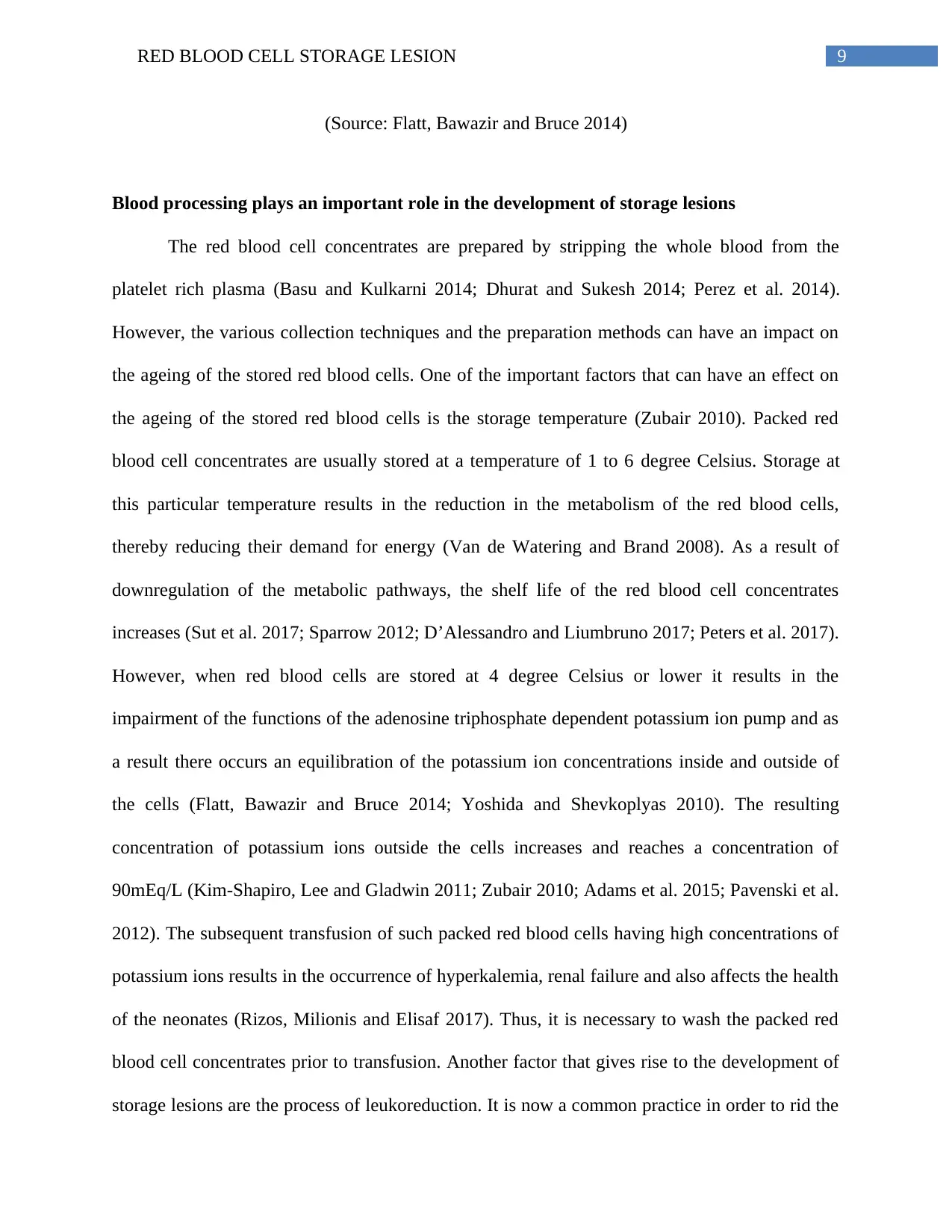
9RED BLOOD CELL STORAGE LESION
(Source: Flatt, Bawazir and Bruce 2014)
Blood processing plays an important role in the development of storage lesions
The red blood cell concentrates are prepared by stripping the whole blood from the
platelet rich plasma (Basu and Kulkarni 2014; Dhurat and Sukesh 2014; Perez et al. 2014).
However, the various collection techniques and the preparation methods can have an impact on
the ageing of the stored red blood cells. One of the important factors that can have an effect on
the ageing of the stored red blood cells is the storage temperature (Zubair 2010). Packed red
blood cell concentrates are usually stored at a temperature of 1 to 6 degree Celsius. Storage at
this particular temperature results in the reduction in the metabolism of the red blood cells,
thereby reducing their demand for energy (Van de Watering and Brand 2008). As a result of
downregulation of the metabolic pathways, the shelf life of the red blood cell concentrates
increases (Sut et al. 2017; Sparrow 2012; D’Alessandro and Liumbruno 2017; Peters et al. 2017).
However, when red blood cells are stored at 4 degree Celsius or lower it results in the
impairment of the functions of the adenosine triphosphate dependent potassium ion pump and as
a result there occurs an equilibration of the potassium ion concentrations inside and outside of
the cells (Flatt, Bawazir and Bruce 2014; Yoshida and Shevkoplyas 2010). The resulting
concentration of potassium ions outside the cells increases and reaches a concentration of
90mEq/L (Kim‐Shapiro, Lee and Gladwin 2011; Zubair 2010; Adams et al. 2015; Pavenski et al.
2012). The subsequent transfusion of such packed red blood cells having high concentrations of
potassium ions results in the occurrence of hyperkalemia, renal failure and also affects the health
of the neonates (Rizos, Milionis and Elisaf 2017). Thus, it is necessary to wash the packed red
blood cell concentrates prior to transfusion. Another factor that gives rise to the development of
storage lesions are the process of leukoreduction. It is now a common practice in order to rid the
(Source: Flatt, Bawazir and Bruce 2014)
Blood processing plays an important role in the development of storage lesions
The red blood cell concentrates are prepared by stripping the whole blood from the
platelet rich plasma (Basu and Kulkarni 2014; Dhurat and Sukesh 2014; Perez et al. 2014).
However, the various collection techniques and the preparation methods can have an impact on
the ageing of the stored red blood cells. One of the important factors that can have an effect on
the ageing of the stored red blood cells is the storage temperature (Zubair 2010). Packed red
blood cell concentrates are usually stored at a temperature of 1 to 6 degree Celsius. Storage at
this particular temperature results in the reduction in the metabolism of the red blood cells,
thereby reducing their demand for energy (Van de Watering and Brand 2008). As a result of
downregulation of the metabolic pathways, the shelf life of the red blood cell concentrates
increases (Sut et al. 2017; Sparrow 2012; D’Alessandro and Liumbruno 2017; Peters et al. 2017).
However, when red blood cells are stored at 4 degree Celsius or lower it results in the
impairment of the functions of the adenosine triphosphate dependent potassium ion pump and as
a result there occurs an equilibration of the potassium ion concentrations inside and outside of
the cells (Flatt, Bawazir and Bruce 2014; Yoshida and Shevkoplyas 2010). The resulting
concentration of potassium ions outside the cells increases and reaches a concentration of
90mEq/L (Kim‐Shapiro, Lee and Gladwin 2011; Zubair 2010; Adams et al. 2015; Pavenski et al.
2012). The subsequent transfusion of such packed red blood cells having high concentrations of
potassium ions results in the occurrence of hyperkalemia, renal failure and also affects the health
of the neonates (Rizos, Milionis and Elisaf 2017). Thus, it is necessary to wash the packed red
blood cell concentrates prior to transfusion. Another factor that gives rise to the development of
storage lesions are the process of leukoreduction. It is now a common practice in order to rid the
Secure Best Marks with AI Grader
Need help grading? Try our AI Grader for instant feedback on your assignments.
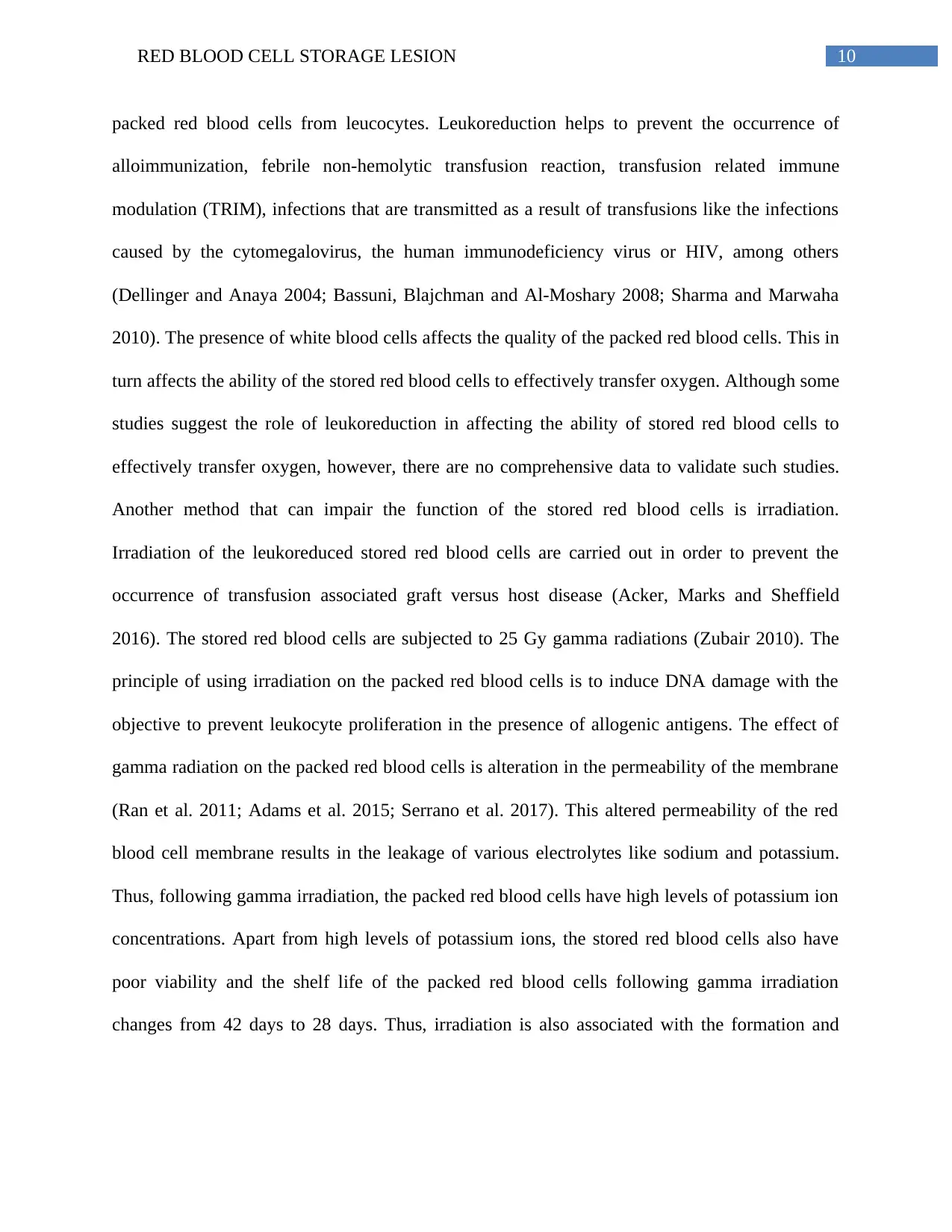
10RED BLOOD CELL STORAGE LESION
packed red blood cells from leucocytes. Leukoreduction helps to prevent the occurrence of
alloimmunization, febrile non-hemolytic transfusion reaction, transfusion related immune
modulation (TRIM), infections that are transmitted as a result of transfusions like the infections
caused by the cytomegalovirus, the human immunodeficiency virus or HIV, among others
(Dellinger and Anaya 2004; Bassuni, Blajchman and Al-Moshary 2008; Sharma and Marwaha
2010). The presence of white blood cells affects the quality of the packed red blood cells. This in
turn affects the ability of the stored red blood cells to effectively transfer oxygen. Although some
studies suggest the role of leukoreduction in affecting the ability of stored red blood cells to
effectively transfer oxygen, however, there are no comprehensive data to validate such studies.
Another method that can impair the function of the stored red blood cells is irradiation.
Irradiation of the leukoreduced stored red blood cells are carried out in order to prevent the
occurrence of transfusion associated graft versus host disease (Acker, Marks and Sheffield
2016). The stored red blood cells are subjected to 25 Gy gamma radiations (Zubair 2010). The
principle of using irradiation on the packed red blood cells is to induce DNA damage with the
objective to prevent leukocyte proliferation in the presence of allogenic antigens. The effect of
gamma radiation on the packed red blood cells is alteration in the permeability of the membrane
(Ran et al. 2011; Adams et al. 2015; Serrano et al. 2017). This altered permeability of the red
blood cell membrane results in the leakage of various electrolytes like sodium and potassium.
Thus, following gamma irradiation, the packed red blood cells have high levels of potassium ion
concentrations. Apart from high levels of potassium ions, the stored red blood cells also have
poor viability and the shelf life of the packed red blood cells following gamma irradiation
changes from 42 days to 28 days. Thus, irradiation is also associated with the formation and
packed red blood cells from leucocytes. Leukoreduction helps to prevent the occurrence of
alloimmunization, febrile non-hemolytic transfusion reaction, transfusion related immune
modulation (TRIM), infections that are transmitted as a result of transfusions like the infections
caused by the cytomegalovirus, the human immunodeficiency virus or HIV, among others
(Dellinger and Anaya 2004; Bassuni, Blajchman and Al-Moshary 2008; Sharma and Marwaha
2010). The presence of white blood cells affects the quality of the packed red blood cells. This in
turn affects the ability of the stored red blood cells to effectively transfer oxygen. Although some
studies suggest the role of leukoreduction in affecting the ability of stored red blood cells to
effectively transfer oxygen, however, there are no comprehensive data to validate such studies.
Another method that can impair the function of the stored red blood cells is irradiation.
Irradiation of the leukoreduced stored red blood cells are carried out in order to prevent the
occurrence of transfusion associated graft versus host disease (Acker, Marks and Sheffield
2016). The stored red blood cells are subjected to 25 Gy gamma radiations (Zubair 2010). The
principle of using irradiation on the packed red blood cells is to induce DNA damage with the
objective to prevent leukocyte proliferation in the presence of allogenic antigens. The effect of
gamma radiation on the packed red blood cells is alteration in the permeability of the membrane
(Ran et al. 2011; Adams et al. 2015; Serrano et al. 2017). This altered permeability of the red
blood cell membrane results in the leakage of various electrolytes like sodium and potassium.
Thus, following gamma irradiation, the packed red blood cells have high levels of potassium ion
concentrations. Apart from high levels of potassium ions, the stored red blood cells also have
poor viability and the shelf life of the packed red blood cells following gamma irradiation
changes from 42 days to 28 days. Thus, irradiation is also associated with the formation and
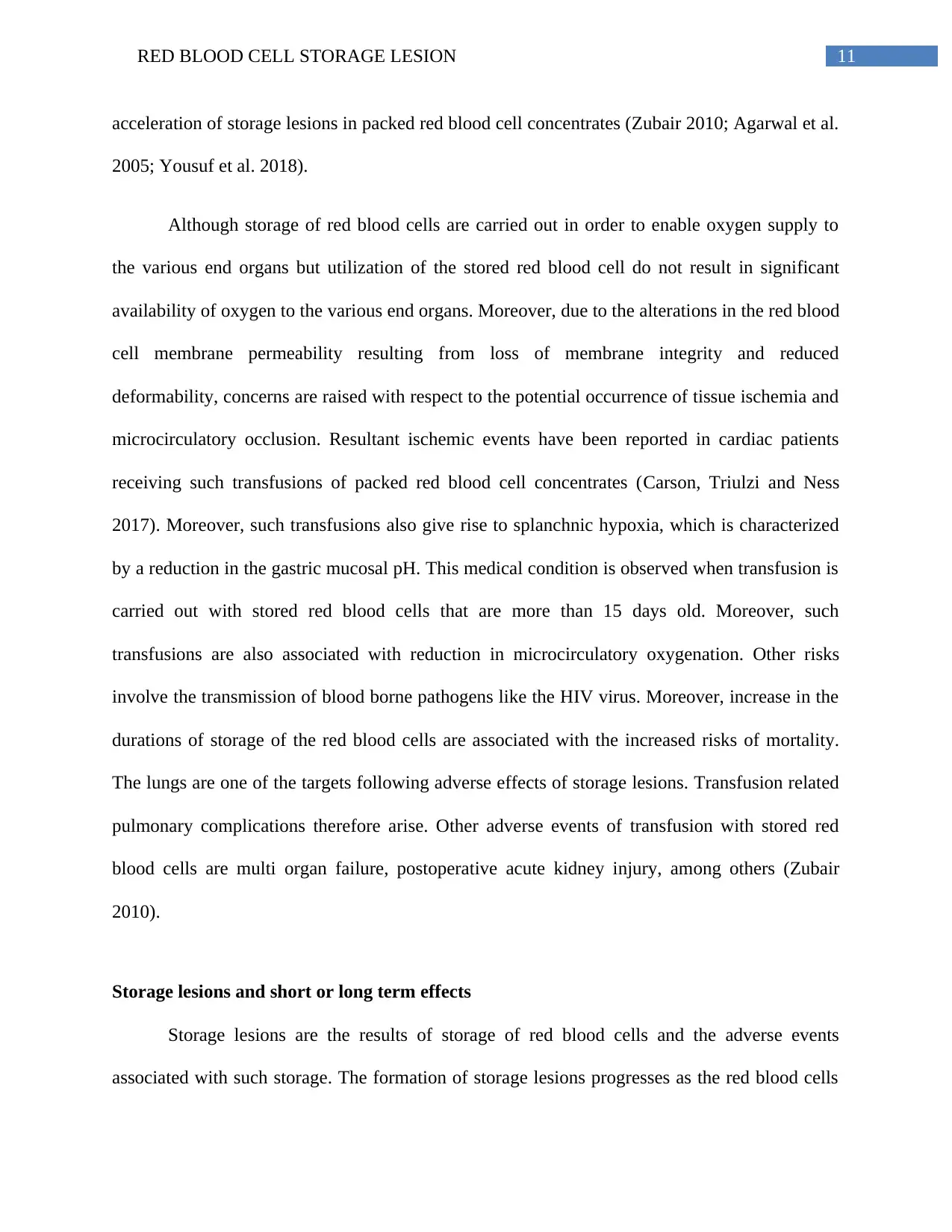
11RED BLOOD CELL STORAGE LESION
acceleration of storage lesions in packed red blood cell concentrates (Zubair 2010; Agarwal et al.
2005; Yousuf et al. 2018).
Although storage of red blood cells are carried out in order to enable oxygen supply to
the various end organs but utilization of the stored red blood cell do not result in significant
availability of oxygen to the various end organs. Moreover, due to the alterations in the red blood
cell membrane permeability resulting from loss of membrane integrity and reduced
deformability, concerns are raised with respect to the potential occurrence of tissue ischemia and
microcirculatory occlusion. Resultant ischemic events have been reported in cardiac patients
receiving such transfusions of packed red blood cell concentrates (Carson, Triulzi and Ness
2017). Moreover, such transfusions also give rise to splanchnic hypoxia, which is characterized
by a reduction in the gastric mucosal pH. This medical condition is observed when transfusion is
carried out with stored red blood cells that are more than 15 days old. Moreover, such
transfusions are also associated with reduction in microcirculatory oxygenation. Other risks
involve the transmission of blood borne pathogens like the HIV virus. Moreover, increase in the
durations of storage of the red blood cells are associated with the increased risks of mortality.
The lungs are one of the targets following adverse effects of storage lesions. Transfusion related
pulmonary complications therefore arise. Other adverse events of transfusion with stored red
blood cells are multi organ failure, postoperative acute kidney injury, among others (Zubair
2010).
Storage lesions and short or long term effects
Storage lesions are the results of storage of red blood cells and the adverse events
associated with such storage. The formation of storage lesions progresses as the red blood cells
acceleration of storage lesions in packed red blood cell concentrates (Zubair 2010; Agarwal et al.
2005; Yousuf et al. 2018).
Although storage of red blood cells are carried out in order to enable oxygen supply to
the various end organs but utilization of the stored red blood cell do not result in significant
availability of oxygen to the various end organs. Moreover, due to the alterations in the red blood
cell membrane permeability resulting from loss of membrane integrity and reduced
deformability, concerns are raised with respect to the potential occurrence of tissue ischemia and
microcirculatory occlusion. Resultant ischemic events have been reported in cardiac patients
receiving such transfusions of packed red blood cell concentrates (Carson, Triulzi and Ness
2017). Moreover, such transfusions also give rise to splanchnic hypoxia, which is characterized
by a reduction in the gastric mucosal pH. This medical condition is observed when transfusion is
carried out with stored red blood cells that are more than 15 days old. Moreover, such
transfusions are also associated with reduction in microcirculatory oxygenation. Other risks
involve the transmission of blood borne pathogens like the HIV virus. Moreover, increase in the
durations of storage of the red blood cells are associated with the increased risks of mortality.
The lungs are one of the targets following adverse effects of storage lesions. Transfusion related
pulmonary complications therefore arise. Other adverse events of transfusion with stored red
blood cells are multi organ failure, postoperative acute kidney injury, among others (Zubair
2010).
Storage lesions and short or long term effects
Storage lesions are the results of storage of red blood cells and the adverse events
associated with such storage. The formation of storage lesions progresses as the red blood cells
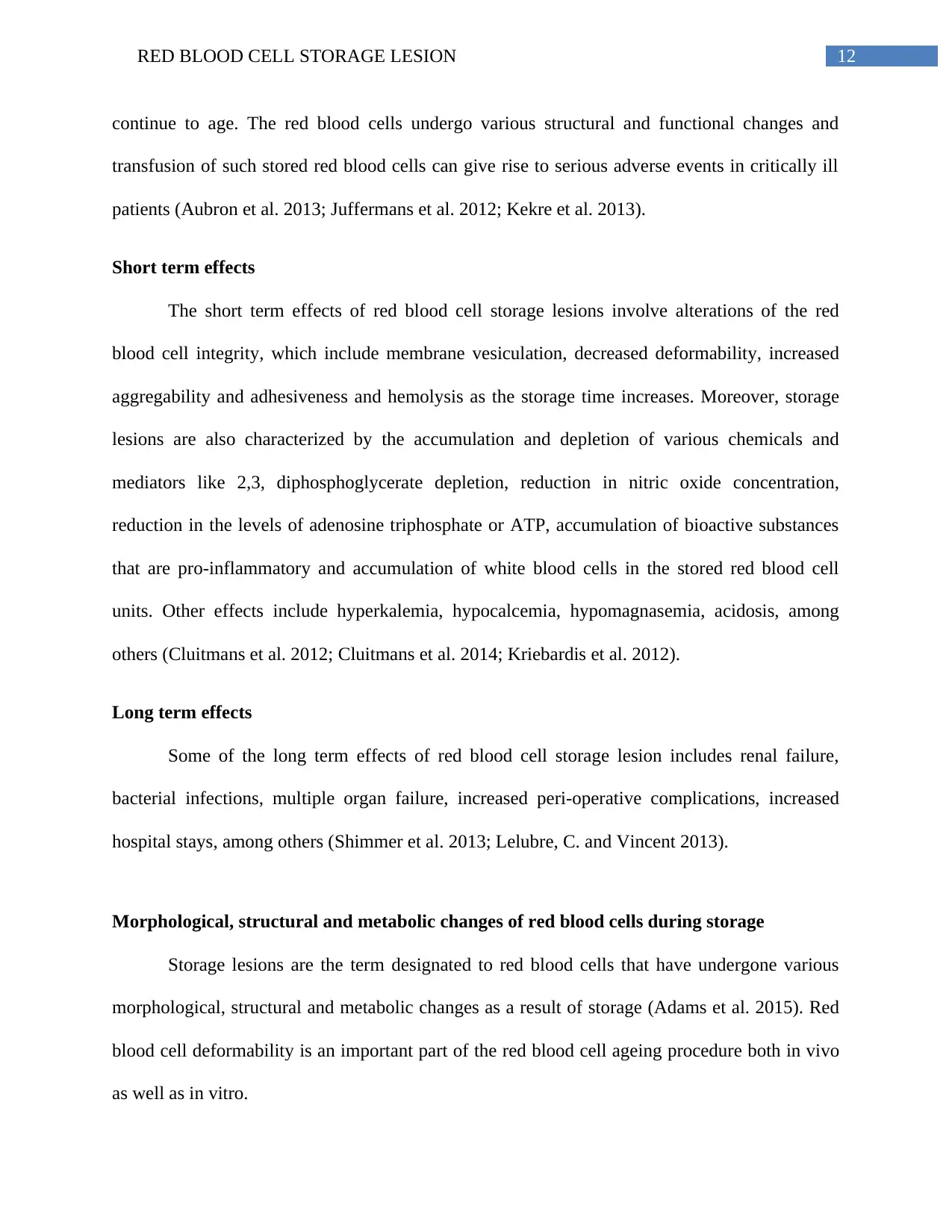
12RED BLOOD CELL STORAGE LESION
continue to age. The red blood cells undergo various structural and functional changes and
transfusion of such stored red blood cells can give rise to serious adverse events in critically ill
patients (Aubron et al. 2013; Juffermans et al. 2012; Kekre et al. 2013).
Short term effects
The short term effects of red blood cell storage lesions involve alterations of the red
blood cell integrity, which include membrane vesiculation, decreased deformability, increased
aggregability and adhesiveness and hemolysis as the storage time increases. Moreover, storage
lesions are also characterized by the accumulation and depletion of various chemicals and
mediators like 2,3, diphosphoglycerate depletion, reduction in nitric oxide concentration,
reduction in the levels of adenosine triphosphate or ATP, accumulation of bioactive substances
that are pro-inflammatory and accumulation of white blood cells in the stored red blood cell
units. Other effects include hyperkalemia, hypocalcemia, hypomagnasemia, acidosis, among
others (Cluitmans et al. 2012; Cluitmans et al. 2014; Kriebardis et al. 2012).
Long term effects
Some of the long term effects of red blood cell storage lesion includes renal failure,
bacterial infections, multiple organ failure, increased peri-operative complications, increased
hospital stays, among others (Shimmer et al. 2013; Lelubre, C. and Vincent 2013).
Morphological, structural and metabolic changes of red blood cells during storage
Storage lesions are the term designated to red blood cells that have undergone various
morphological, structural and metabolic changes as a result of storage (Adams et al. 2015). Red
blood cell deformability is an important part of the red blood cell ageing procedure both in vivo
as well as in vitro.
continue to age. The red blood cells undergo various structural and functional changes and
transfusion of such stored red blood cells can give rise to serious adverse events in critically ill
patients (Aubron et al. 2013; Juffermans et al. 2012; Kekre et al. 2013).
Short term effects
The short term effects of red blood cell storage lesions involve alterations of the red
blood cell integrity, which include membrane vesiculation, decreased deformability, increased
aggregability and adhesiveness and hemolysis as the storage time increases. Moreover, storage
lesions are also characterized by the accumulation and depletion of various chemicals and
mediators like 2,3, diphosphoglycerate depletion, reduction in nitric oxide concentration,
reduction in the levels of adenosine triphosphate or ATP, accumulation of bioactive substances
that are pro-inflammatory and accumulation of white blood cells in the stored red blood cell
units. Other effects include hyperkalemia, hypocalcemia, hypomagnasemia, acidosis, among
others (Cluitmans et al. 2012; Cluitmans et al. 2014; Kriebardis et al. 2012).
Long term effects
Some of the long term effects of red blood cell storage lesion includes renal failure,
bacterial infections, multiple organ failure, increased peri-operative complications, increased
hospital stays, among others (Shimmer et al. 2013; Lelubre, C. and Vincent 2013).
Morphological, structural and metabolic changes of red blood cells during storage
Storage lesions are the term designated to red blood cells that have undergone various
morphological, structural and metabolic changes as a result of storage (Adams et al. 2015). Red
blood cell deformability is an important part of the red blood cell ageing procedure both in vivo
as well as in vitro.
Paraphrase This Document
Need a fresh take? Get an instant paraphrase of this document with our AI Paraphraser
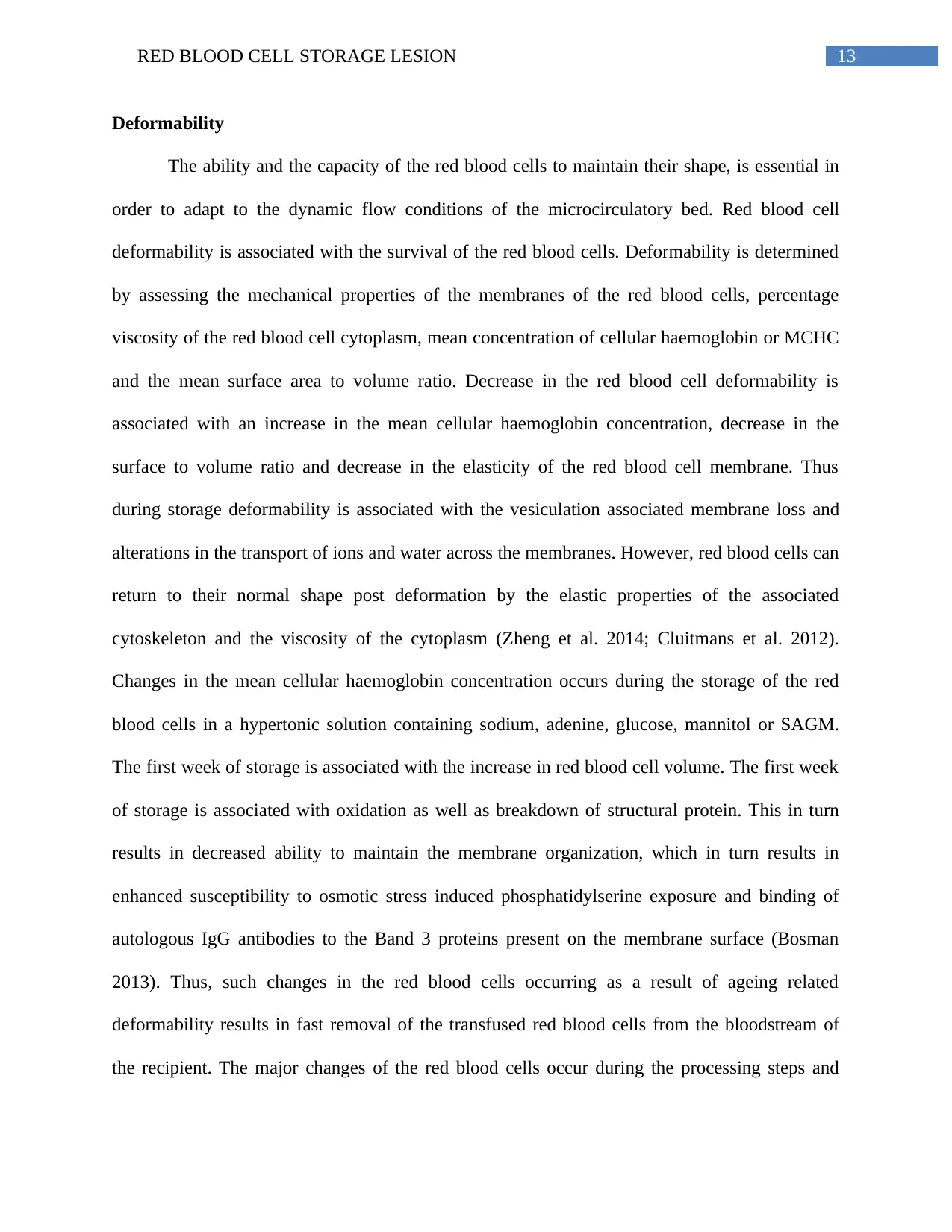
13RED BLOOD CELL STORAGE LESION
Deformability
The ability and the capacity of the red blood cells to maintain their shape, is essential in
order to adapt to the dynamic flow conditions of the microcirculatory bed. Red blood cell
deformability is associated with the survival of the red blood cells. Deformability is determined
by assessing the mechanical properties of the membranes of the red blood cells, percentage
viscosity of the red blood cell cytoplasm, mean concentration of cellular haemoglobin or MCHC
and the mean surface area to volume ratio. Decrease in the red blood cell deformability is
associated with an increase in the mean cellular haemoglobin concentration, decrease in the
surface to volume ratio and decrease in the elasticity of the red blood cell membrane. Thus
during storage deformability is associated with the vesiculation associated membrane loss and
alterations in the transport of ions and water across the membranes. However, red blood cells can
return to their normal shape post deformation by the elastic properties of the associated
cytoskeleton and the viscosity of the cytoplasm (Zheng et al. 2014; Cluitmans et al. 2012).
Changes in the mean cellular haemoglobin concentration occurs during the storage of the red
blood cells in a hypertonic solution containing sodium, adenine, glucose, mannitol or SAGM.
The first week of storage is associated with the increase in red blood cell volume. The first week
of storage is associated with oxidation as well as breakdown of structural protein. This in turn
results in decreased ability to maintain the membrane organization, which in turn results in
enhanced susceptibility to osmotic stress induced phosphatidylserine exposure and binding of
autologous IgG antibodies to the Band 3 proteins present on the membrane surface (Bosman
2013). Thus, such changes in the red blood cells occurring as a result of ageing related
deformability results in fast removal of the transfused red blood cells from the bloodstream of
the recipient. The major changes of the red blood cells occur during the processing steps and
Deformability
The ability and the capacity of the red blood cells to maintain their shape, is essential in
order to adapt to the dynamic flow conditions of the microcirculatory bed. Red blood cell
deformability is associated with the survival of the red blood cells. Deformability is determined
by assessing the mechanical properties of the membranes of the red blood cells, percentage
viscosity of the red blood cell cytoplasm, mean concentration of cellular haemoglobin or MCHC
and the mean surface area to volume ratio. Decrease in the red blood cell deformability is
associated with an increase in the mean cellular haemoglobin concentration, decrease in the
surface to volume ratio and decrease in the elasticity of the red blood cell membrane. Thus
during storage deformability is associated with the vesiculation associated membrane loss and
alterations in the transport of ions and water across the membranes. However, red blood cells can
return to their normal shape post deformation by the elastic properties of the associated
cytoskeleton and the viscosity of the cytoplasm (Zheng et al. 2014; Cluitmans et al. 2012).
Changes in the mean cellular haemoglobin concentration occurs during the storage of the red
blood cells in a hypertonic solution containing sodium, adenine, glucose, mannitol or SAGM.
The first week of storage is associated with the increase in red blood cell volume. The first week
of storage is associated with oxidation as well as breakdown of structural protein. This in turn
results in decreased ability to maintain the membrane organization, which in turn results in
enhanced susceptibility to osmotic stress induced phosphatidylserine exposure and binding of
autologous IgG antibodies to the Band 3 proteins present on the membrane surface (Bosman
2013). Thus, such changes in the red blood cells occurring as a result of ageing related
deformability results in fast removal of the transfused red blood cells from the bloodstream of
the recipient. The major changes of the red blood cells occur during the processing steps and
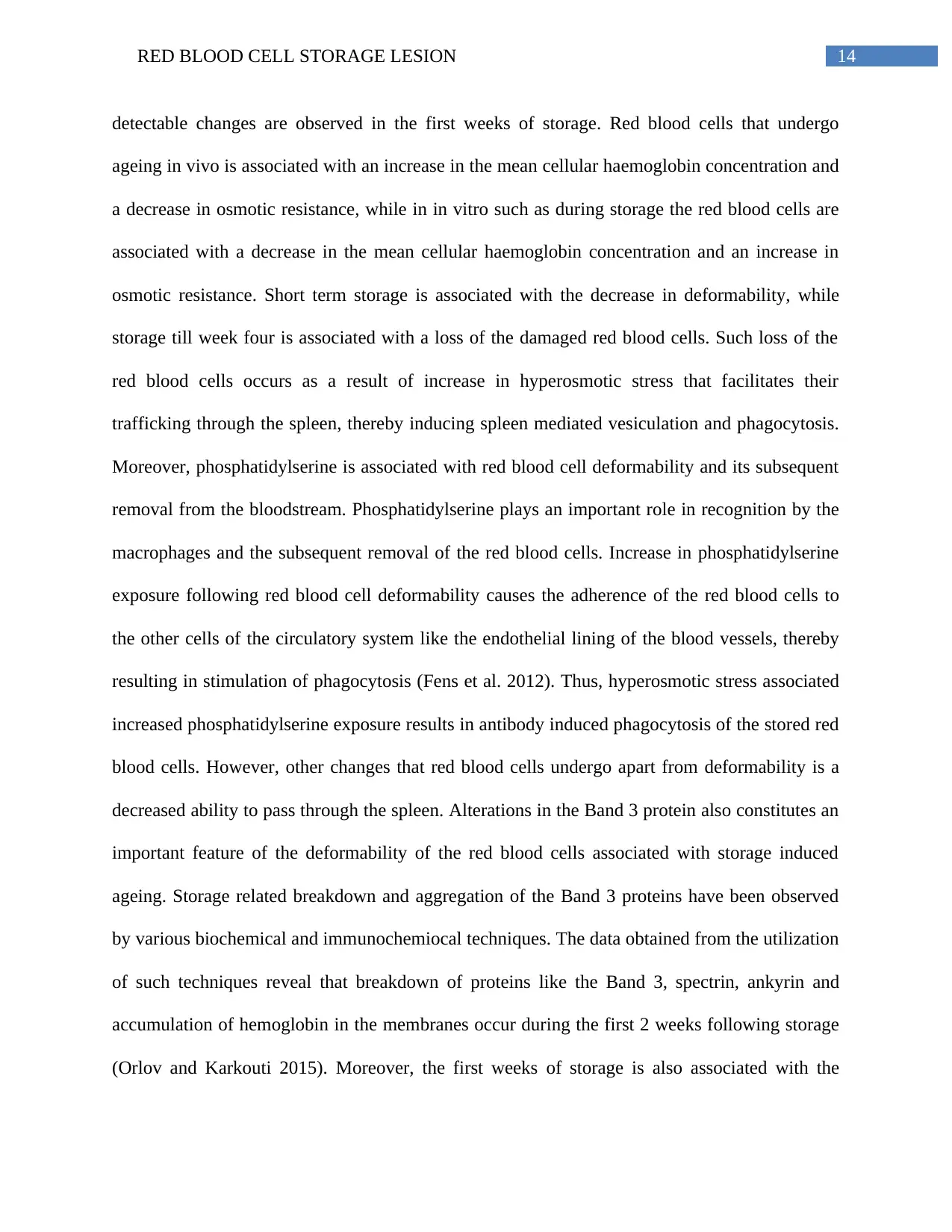
14RED BLOOD CELL STORAGE LESION
detectable changes are observed in the first weeks of storage. Red blood cells that undergo
ageing in vivo is associated with an increase in the mean cellular haemoglobin concentration and
a decrease in osmotic resistance, while in in vitro such as during storage the red blood cells are
associated with a decrease in the mean cellular haemoglobin concentration and an increase in
osmotic resistance. Short term storage is associated with the decrease in deformability, while
storage till week four is associated with a loss of the damaged red blood cells. Such loss of the
red blood cells occurs as a result of increase in hyperosmotic stress that facilitates their
trafficking through the spleen, thereby inducing spleen mediated vesiculation and phagocytosis.
Moreover, phosphatidylserine is associated with red blood cell deformability and its subsequent
removal from the bloodstream. Phosphatidylserine plays an important role in recognition by the
macrophages and the subsequent removal of the red blood cells. Increase in phosphatidylserine
exposure following red blood cell deformability causes the adherence of the red blood cells to
the other cells of the circulatory system like the endothelial lining of the blood vessels, thereby
resulting in stimulation of phagocytosis (Fens et al. 2012). Thus, hyperosmotic stress associated
increased phosphatidylserine exposure results in antibody induced phagocytosis of the stored red
blood cells. However, other changes that red blood cells undergo apart from deformability is a
decreased ability to pass through the spleen. Alterations in the Band 3 protein also constitutes an
important feature of the deformability of the red blood cells associated with storage induced
ageing. Storage related breakdown and aggregation of the Band 3 proteins have been observed
by various biochemical and immunochemiocal techniques. The data obtained from the utilization
of such techniques reveal that breakdown of proteins like the Band 3, spectrin, ankyrin and
accumulation of hemoglobin in the membranes occur during the first 2 weeks following storage
(Orlov and Karkouti 2015). Moreover, the first weeks of storage is also associated with the
detectable changes are observed in the first weeks of storage. Red blood cells that undergo
ageing in vivo is associated with an increase in the mean cellular haemoglobin concentration and
a decrease in osmotic resistance, while in in vitro such as during storage the red blood cells are
associated with a decrease in the mean cellular haemoglobin concentration and an increase in
osmotic resistance. Short term storage is associated with the decrease in deformability, while
storage till week four is associated with a loss of the damaged red blood cells. Such loss of the
red blood cells occurs as a result of increase in hyperosmotic stress that facilitates their
trafficking through the spleen, thereby inducing spleen mediated vesiculation and phagocytosis.
Moreover, phosphatidylserine is associated with red blood cell deformability and its subsequent
removal from the bloodstream. Phosphatidylserine plays an important role in recognition by the
macrophages and the subsequent removal of the red blood cells. Increase in phosphatidylserine
exposure following red blood cell deformability causes the adherence of the red blood cells to
the other cells of the circulatory system like the endothelial lining of the blood vessels, thereby
resulting in stimulation of phagocytosis (Fens et al. 2012). Thus, hyperosmotic stress associated
increased phosphatidylserine exposure results in antibody induced phagocytosis of the stored red
blood cells. However, other changes that red blood cells undergo apart from deformability is a
decreased ability to pass through the spleen. Alterations in the Band 3 protein also constitutes an
important feature of the deformability of the red blood cells associated with storage induced
ageing. Storage related breakdown and aggregation of the Band 3 proteins have been observed
by various biochemical and immunochemiocal techniques. The data obtained from the utilization
of such techniques reveal that breakdown of proteins like the Band 3, spectrin, ankyrin and
accumulation of hemoglobin in the membranes occur during the first 2 weeks following storage
(Orlov and Karkouti 2015). Moreover, the first weeks of storage is also associated with the
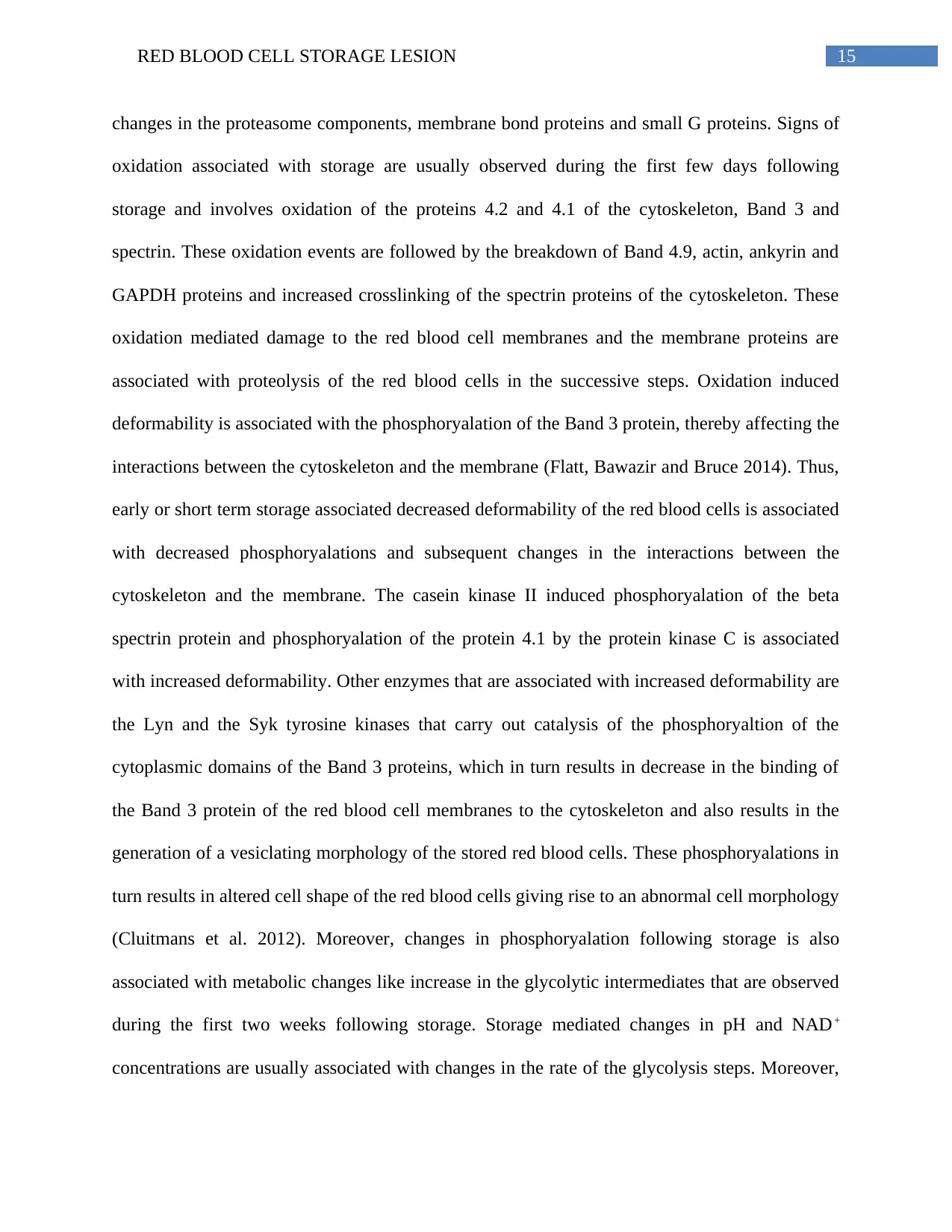
15RED BLOOD CELL STORAGE LESION
changes in the proteasome components, membrane bond proteins and small G proteins. Signs of
oxidation associated with storage are usually observed during the first few days following
storage and involves oxidation of the proteins 4.2 and 4.1 of the cytoskeleton, Band 3 and
spectrin. These oxidation events are followed by the breakdown of Band 4.9, actin, ankyrin and
GAPDH proteins and increased crosslinking of the spectrin proteins of the cytoskeleton. These
oxidation mediated damage to the red blood cell membranes and the membrane proteins are
associated with proteolysis of the red blood cells in the successive steps. Oxidation induced
deformability is associated with the phosphoryalation of the Band 3 protein, thereby affecting the
interactions between the cytoskeleton and the membrane (Flatt, Bawazir and Bruce 2014). Thus,
early or short term storage associated decreased deformability of the red blood cells is associated
with decreased phosphoryalations and subsequent changes in the interactions between the
cytoskeleton and the membrane. The casein kinase II induced phosphoryalation of the beta
spectrin protein and phosphoryalation of the protein 4.1 by the protein kinase C is associated
with increased deformability. Other enzymes that are associated with increased deformability are
the Lyn and the Syk tyrosine kinases that carry out catalysis of the phosphoryaltion of the
cytoplasmic domains of the Band 3 proteins, which in turn results in decrease in the binding of
the Band 3 protein of the red blood cell membranes to the cytoskeleton and also results in the
generation of a vesiclating morphology of the stored red blood cells. These phosphoryalations in
turn results in altered cell shape of the red blood cells giving rise to an abnormal cell morphology
(Cluitmans et al. 2012). Moreover, changes in phosphoryalation following storage is also
associated with metabolic changes like increase in the glycolytic intermediates that are observed
during the first two weeks following storage. Storage mediated changes in pH and NAD+
concentrations are usually associated with changes in the rate of the glycolysis steps. Moreover,
changes in the proteasome components, membrane bond proteins and small G proteins. Signs of
oxidation associated with storage are usually observed during the first few days following
storage and involves oxidation of the proteins 4.2 and 4.1 of the cytoskeleton, Band 3 and
spectrin. These oxidation events are followed by the breakdown of Band 4.9, actin, ankyrin and
GAPDH proteins and increased crosslinking of the spectrin proteins of the cytoskeleton. These
oxidation mediated damage to the red blood cell membranes and the membrane proteins are
associated with proteolysis of the red blood cells in the successive steps. Oxidation induced
deformability is associated with the phosphoryalation of the Band 3 protein, thereby affecting the
interactions between the cytoskeleton and the membrane (Flatt, Bawazir and Bruce 2014). Thus,
early or short term storage associated decreased deformability of the red blood cells is associated
with decreased phosphoryalations and subsequent changes in the interactions between the
cytoskeleton and the membrane. The casein kinase II induced phosphoryalation of the beta
spectrin protein and phosphoryalation of the protein 4.1 by the protein kinase C is associated
with increased deformability. Other enzymes that are associated with increased deformability are
the Lyn and the Syk tyrosine kinases that carry out catalysis of the phosphoryaltion of the
cytoplasmic domains of the Band 3 proteins, which in turn results in decrease in the binding of
the Band 3 protein of the red blood cell membranes to the cytoskeleton and also results in the
generation of a vesiclating morphology of the stored red blood cells. These phosphoryalations in
turn results in altered cell shape of the red blood cells giving rise to an abnormal cell morphology
(Cluitmans et al. 2012). Moreover, changes in phosphoryalation following storage is also
associated with metabolic changes like increase in the glycolytic intermediates that are observed
during the first two weeks following storage. Storage mediated changes in pH and NAD+
concentrations are usually associated with changes in the rate of the glycolysis steps. Moreover,
Secure Best Marks with AI Grader
Need help grading? Try our AI Grader for instant feedback on your assignments.
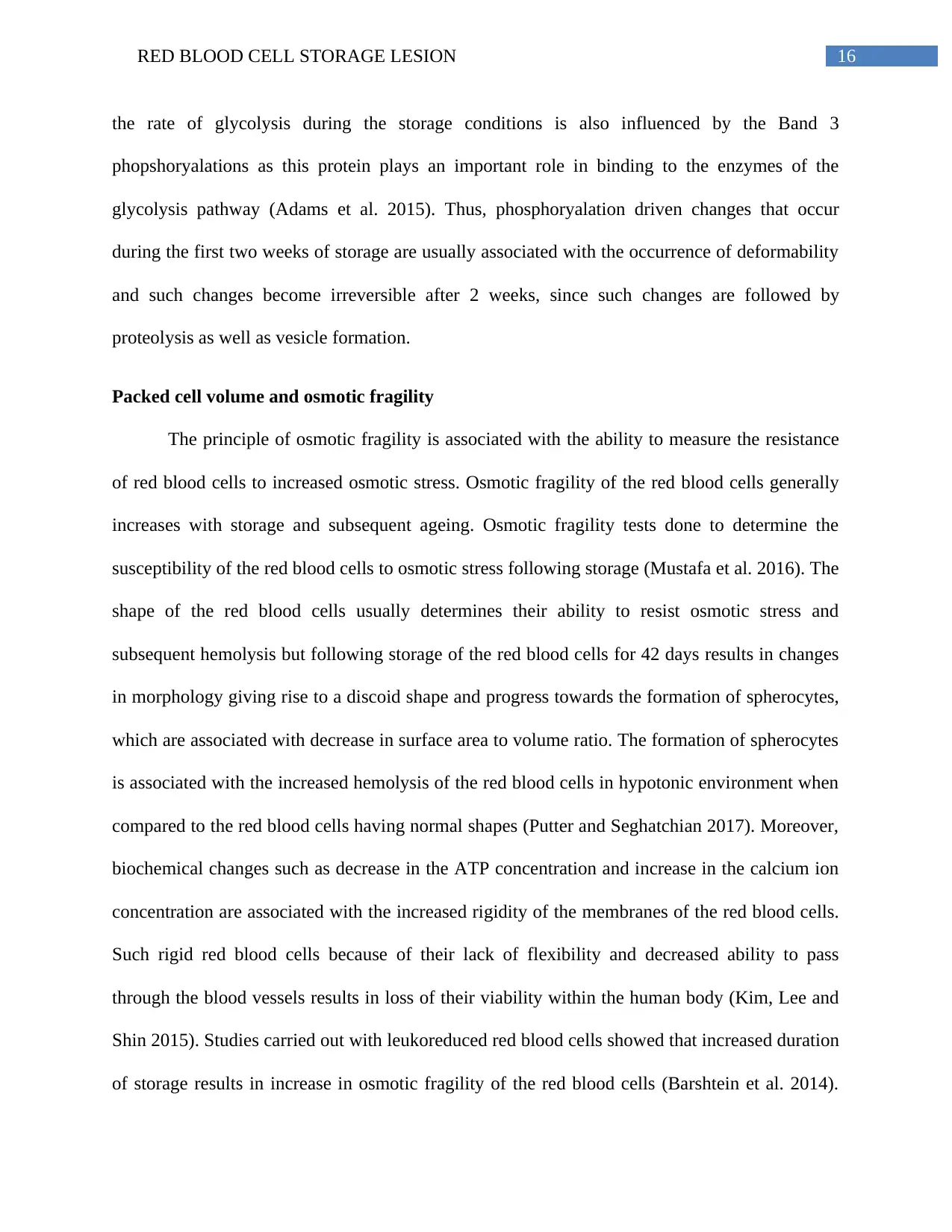
16RED BLOOD CELL STORAGE LESION
the rate of glycolysis during the storage conditions is also influenced by the Band 3
phopshoryalations as this protein plays an important role in binding to the enzymes of the
glycolysis pathway (Adams et al. 2015). Thus, phosphoryalation driven changes that occur
during the first two weeks of storage are usually associated with the occurrence of deformability
and such changes become irreversible after 2 weeks, since such changes are followed by
proteolysis as well as vesicle formation.
Packed cell volume and osmotic fragility
The principle of osmotic fragility is associated with the ability to measure the resistance
of red blood cells to increased osmotic stress. Osmotic fragility of the red blood cells generally
increases with storage and subsequent ageing. Osmotic fragility tests done to determine the
susceptibility of the red blood cells to osmotic stress following storage (Mustafa et al. 2016). The
shape of the red blood cells usually determines their ability to resist osmotic stress and
subsequent hemolysis but following storage of the red blood cells for 42 days results in changes
in morphology giving rise to a discoid shape and progress towards the formation of spherocytes,
which are associated with decrease in surface area to volume ratio. The formation of spherocytes
is associated with the increased hemolysis of the red blood cells in hypotonic environment when
compared to the red blood cells having normal shapes (Putter and Seghatchian 2017). Moreover,
biochemical changes such as decrease in the ATP concentration and increase in the calcium ion
concentration are associated with the increased rigidity of the membranes of the red blood cells.
Such rigid red blood cells because of their lack of flexibility and decreased ability to pass
through the blood vessels results in loss of their viability within the human body (Kim, Lee and
Shin 2015). Studies carried out with leukoreduced red blood cells showed that increased duration
of storage results in increase in osmotic fragility of the red blood cells (Barshtein et al. 2014).
the rate of glycolysis during the storage conditions is also influenced by the Band 3
phopshoryalations as this protein plays an important role in binding to the enzymes of the
glycolysis pathway (Adams et al. 2015). Thus, phosphoryalation driven changes that occur
during the first two weeks of storage are usually associated with the occurrence of deformability
and such changes become irreversible after 2 weeks, since such changes are followed by
proteolysis as well as vesicle formation.
Packed cell volume and osmotic fragility
The principle of osmotic fragility is associated with the ability to measure the resistance
of red blood cells to increased osmotic stress. Osmotic fragility of the red blood cells generally
increases with storage and subsequent ageing. Osmotic fragility tests done to determine the
susceptibility of the red blood cells to osmotic stress following storage (Mustafa et al. 2016). The
shape of the red blood cells usually determines their ability to resist osmotic stress and
subsequent hemolysis but following storage of the red blood cells for 42 days results in changes
in morphology giving rise to a discoid shape and progress towards the formation of spherocytes,
which are associated with decrease in surface area to volume ratio. The formation of spherocytes
is associated with the increased hemolysis of the red blood cells in hypotonic environment when
compared to the red blood cells having normal shapes (Putter and Seghatchian 2017). Moreover,
biochemical changes such as decrease in the ATP concentration and increase in the calcium ion
concentration are associated with the increased rigidity of the membranes of the red blood cells.
Such rigid red blood cells because of their lack of flexibility and decreased ability to pass
through the blood vessels results in loss of their viability within the human body (Kim, Lee and
Shin 2015). Studies carried out with leukoreduced red blood cells showed that increased duration
of storage results in increase in osmotic fragility of the red blood cells (Barshtein et al. 2014).
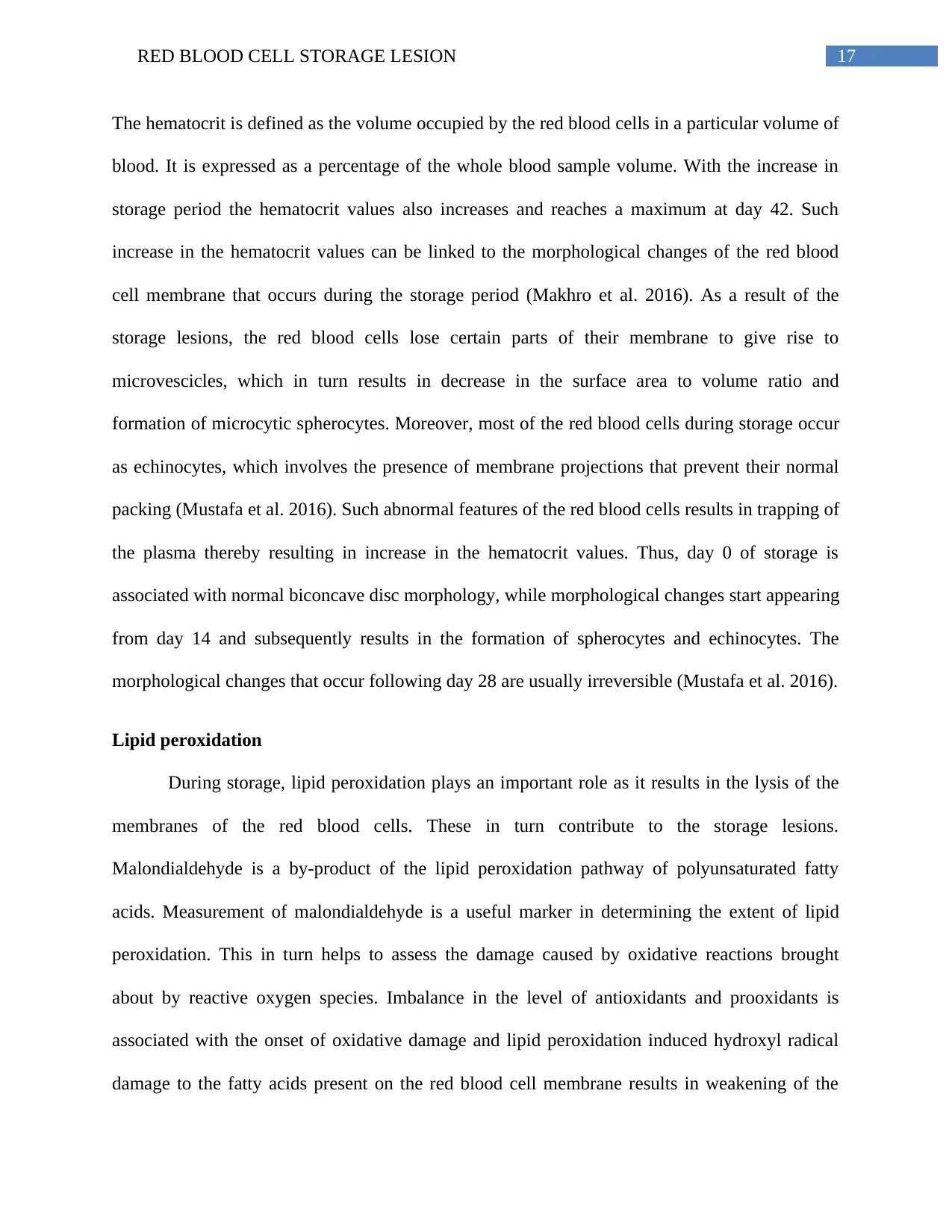
17RED BLOOD CELL STORAGE LESION
The hematocrit is defined as the volume occupied by the red blood cells in a particular volume of
blood. It is expressed as a percentage of the whole blood sample volume. With the increase in
storage period the hematocrit values also increases and reaches a maximum at day 42. Such
increase in the hematocrit values can be linked to the morphological changes of the red blood
cell membrane that occurs during the storage period (Makhro et al. 2016). As a result of the
storage lesions, the red blood cells lose certain parts of their membrane to give rise to
microvescicles, which in turn results in decrease in the surface area to volume ratio and
formation of microcytic spherocytes. Moreover, most of the red blood cells during storage occur
as echinocytes, which involves the presence of membrane projections that prevent their normal
packing (Mustafa et al. 2016). Such abnormal features of the red blood cells results in trapping of
the plasma thereby resulting in increase in the hematocrit values. Thus, day 0 of storage is
associated with normal biconcave disc morphology, while morphological changes start appearing
from day 14 and subsequently results in the formation of spherocytes and echinocytes. The
morphological changes that occur following day 28 are usually irreversible (Mustafa et al. 2016).
Lipid peroxidation
During storage, lipid peroxidation plays an important role as it results in the lysis of the
membranes of the red blood cells. These in turn contribute to the storage lesions.
Malondialdehyde is a by-product of the lipid peroxidation pathway of polyunsaturated fatty
acids. Measurement of malondialdehyde is a useful marker in determining the extent of lipid
peroxidation. This in turn helps to assess the damage caused by oxidative reactions brought
about by reactive oxygen species. Imbalance in the level of antioxidants and prooxidants is
associated with the onset of oxidative damage and lipid peroxidation induced hydroxyl radical
damage to the fatty acids present on the red blood cell membrane results in weakening of the
The hematocrit is defined as the volume occupied by the red blood cells in a particular volume of
blood. It is expressed as a percentage of the whole blood sample volume. With the increase in
storage period the hematocrit values also increases and reaches a maximum at day 42. Such
increase in the hematocrit values can be linked to the morphological changes of the red blood
cell membrane that occurs during the storage period (Makhro et al. 2016). As a result of the
storage lesions, the red blood cells lose certain parts of their membrane to give rise to
microvescicles, which in turn results in decrease in the surface area to volume ratio and
formation of microcytic spherocytes. Moreover, most of the red blood cells during storage occur
as echinocytes, which involves the presence of membrane projections that prevent their normal
packing (Mustafa et al. 2016). Such abnormal features of the red blood cells results in trapping of
the plasma thereby resulting in increase in the hematocrit values. Thus, day 0 of storage is
associated with normal biconcave disc morphology, while morphological changes start appearing
from day 14 and subsequently results in the formation of spherocytes and echinocytes. The
morphological changes that occur following day 28 are usually irreversible (Mustafa et al. 2016).
Lipid peroxidation
During storage, lipid peroxidation plays an important role as it results in the lysis of the
membranes of the red blood cells. These in turn contribute to the storage lesions.
Malondialdehyde is a by-product of the lipid peroxidation pathway of polyunsaturated fatty
acids. Measurement of malondialdehyde is a useful marker in determining the extent of lipid
peroxidation. This in turn helps to assess the damage caused by oxidative reactions brought
about by reactive oxygen species. Imbalance in the level of antioxidants and prooxidants is
associated with the onset of oxidative damage and lipid peroxidation induced hydroxyl radical
damage to the fatty acids present on the red blood cell membrane results in weakening of the
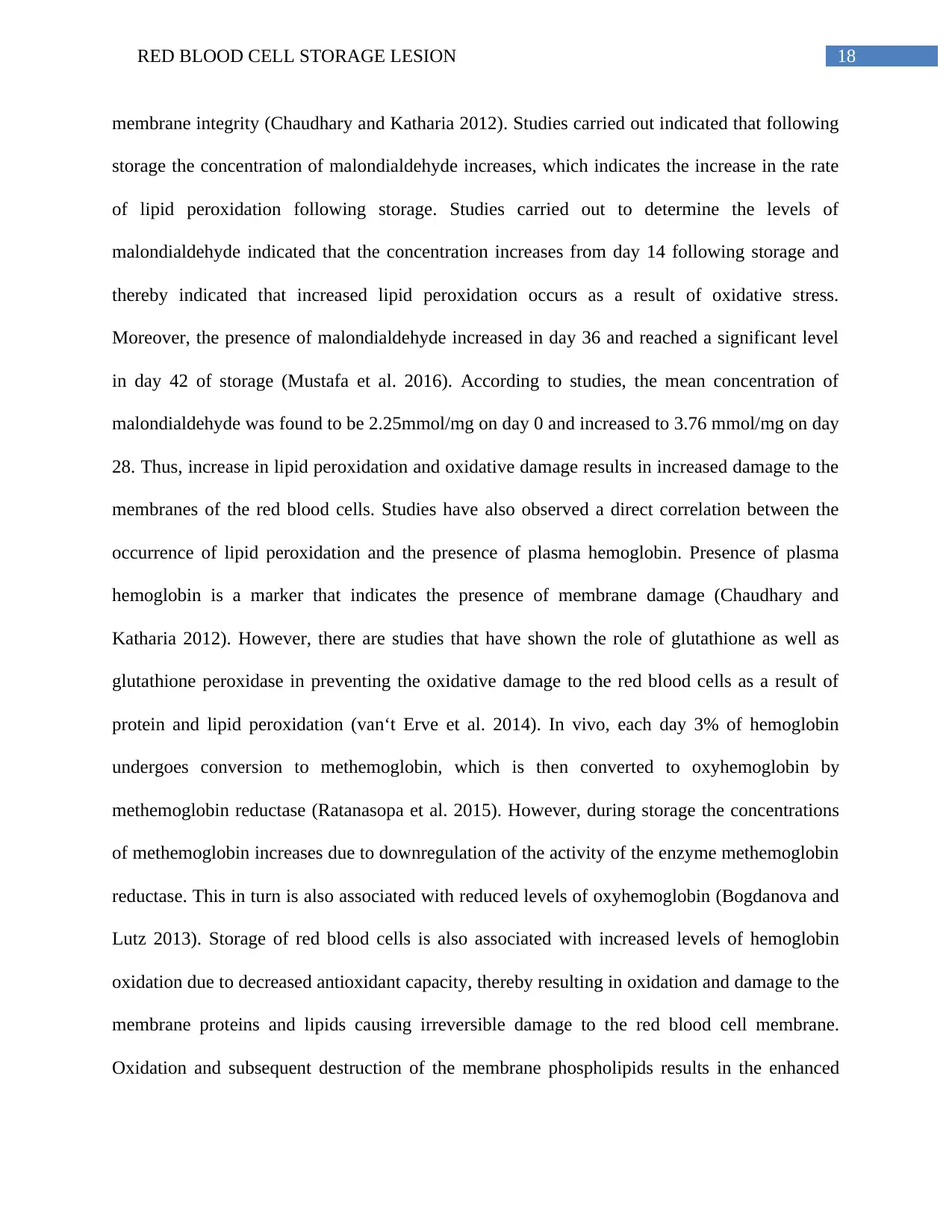
18RED BLOOD CELL STORAGE LESION
membrane integrity (Chaudhary and Katharia 2012). Studies carried out indicated that following
storage the concentration of malondialdehyde increases, which indicates the increase in the rate
of lipid peroxidation following storage. Studies carried out to determine the levels of
malondialdehyde indicated that the concentration increases from day 14 following storage and
thereby indicated that increased lipid peroxidation occurs as a result of oxidative stress.
Moreover, the presence of malondialdehyde increased in day 36 and reached a significant level
in day 42 of storage (Mustafa et al. 2016). According to studies, the mean concentration of
malondialdehyde was found to be 2.25mmol/mg on day 0 and increased to 3.76 mmol/mg on day
28. Thus, increase in lipid peroxidation and oxidative damage results in increased damage to the
membranes of the red blood cells. Studies have also observed a direct correlation between the
occurrence of lipid peroxidation and the presence of plasma hemoglobin. Presence of plasma
hemoglobin is a marker that indicates the presence of membrane damage (Chaudhary and
Katharia 2012). However, there are studies that have shown the role of glutathione as well as
glutathione peroxidase in preventing the oxidative damage to the red blood cells as a result of
protein and lipid peroxidation (van‘t Erve et al. 2014). In vivo, each day 3% of hemoglobin
undergoes conversion to methemoglobin, which is then converted to oxyhemoglobin by
methemoglobin reductase (Ratanasopa et al. 2015). However, during storage the concentrations
of methemoglobin increases due to downregulation of the activity of the enzyme methemoglobin
reductase. This in turn is also associated with reduced levels of oxyhemoglobin (Bogdanova and
Lutz 2013). Storage of red blood cells is also associated with increased levels of hemoglobin
oxidation due to decreased antioxidant capacity, thereby resulting in oxidation and damage to the
membrane proteins and lipids causing irreversible damage to the red blood cell membrane.
Oxidation and subsequent destruction of the membrane phospholipids results in the enhanced
membrane integrity (Chaudhary and Katharia 2012). Studies carried out indicated that following
storage the concentration of malondialdehyde increases, which indicates the increase in the rate
of lipid peroxidation following storage. Studies carried out to determine the levels of
malondialdehyde indicated that the concentration increases from day 14 following storage and
thereby indicated that increased lipid peroxidation occurs as a result of oxidative stress.
Moreover, the presence of malondialdehyde increased in day 36 and reached a significant level
in day 42 of storage (Mustafa et al. 2016). According to studies, the mean concentration of
malondialdehyde was found to be 2.25mmol/mg on day 0 and increased to 3.76 mmol/mg on day
28. Thus, increase in lipid peroxidation and oxidative damage results in increased damage to the
membranes of the red blood cells. Studies have also observed a direct correlation between the
occurrence of lipid peroxidation and the presence of plasma hemoglobin. Presence of plasma
hemoglobin is a marker that indicates the presence of membrane damage (Chaudhary and
Katharia 2012). However, there are studies that have shown the role of glutathione as well as
glutathione peroxidase in preventing the oxidative damage to the red blood cells as a result of
protein and lipid peroxidation (van‘t Erve et al. 2014). In vivo, each day 3% of hemoglobin
undergoes conversion to methemoglobin, which is then converted to oxyhemoglobin by
methemoglobin reductase (Ratanasopa et al. 2015). However, during storage the concentrations
of methemoglobin increases due to downregulation of the activity of the enzyme methemoglobin
reductase. This in turn is also associated with reduced levels of oxyhemoglobin (Bogdanova and
Lutz 2013). Storage of red blood cells is also associated with increased levels of hemoglobin
oxidation due to decreased antioxidant capacity, thereby resulting in oxidation and damage to the
membrane proteins and lipids causing irreversible damage to the red blood cell membrane.
Oxidation and subsequent destruction of the membrane phospholipids results in the enhanced
Paraphrase This Document
Need a fresh take? Get an instant paraphrase of this document with our AI Paraphraser
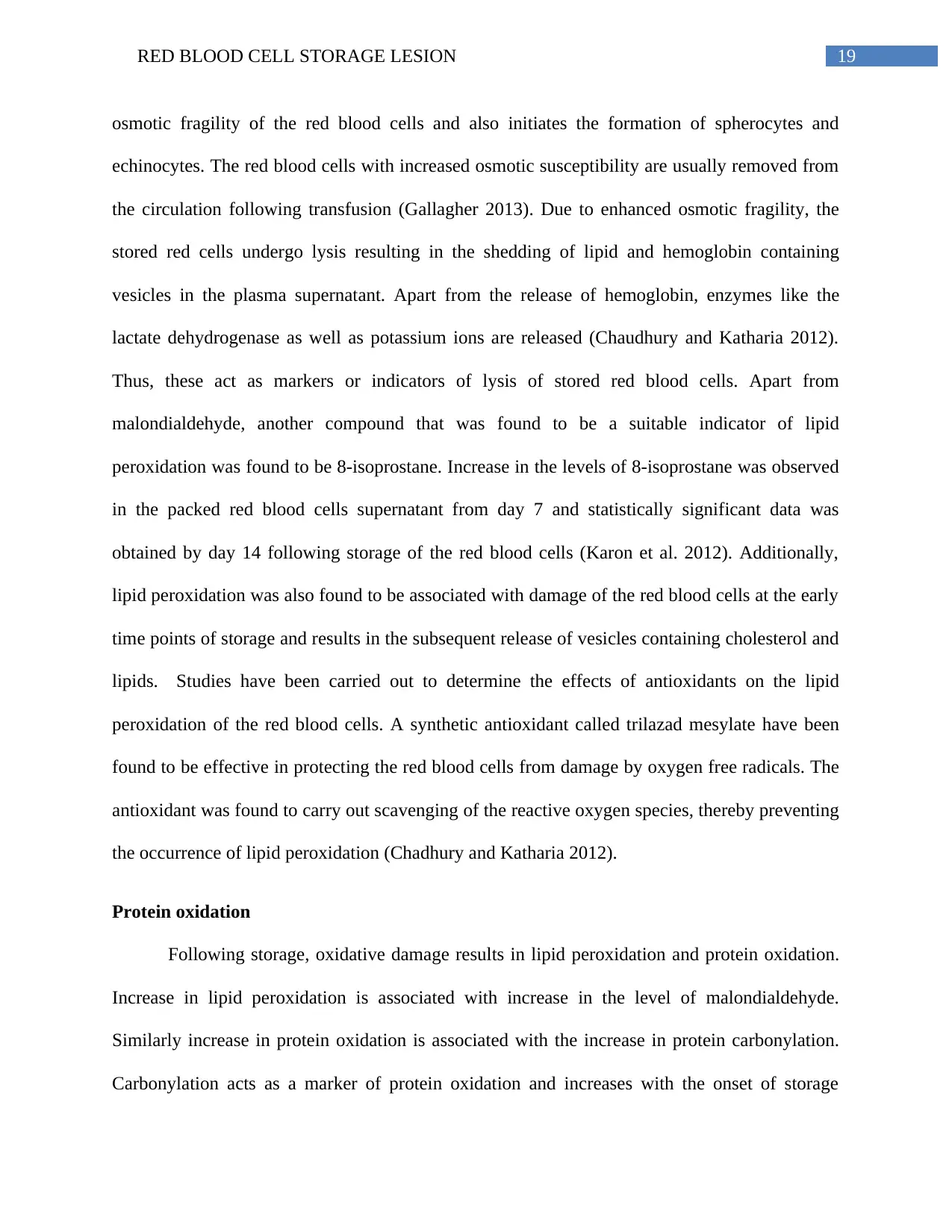
19RED BLOOD CELL STORAGE LESION
osmotic fragility of the red blood cells and also initiates the formation of spherocytes and
echinocytes. The red blood cells with increased osmotic susceptibility are usually removed from
the circulation following transfusion (Gallagher 2013). Due to enhanced osmotic fragility, the
stored red cells undergo lysis resulting in the shedding of lipid and hemoglobin containing
vesicles in the plasma supernatant. Apart from the release of hemoglobin, enzymes like the
lactate dehydrogenase as well as potassium ions are released (Chaudhury and Katharia 2012).
Thus, these act as markers or indicators of lysis of stored red blood cells. Apart from
malondialdehyde, another compound that was found to be a suitable indicator of lipid
peroxidation was found to be 8-isoprostane. Increase in the levels of 8-isoprostane was observed
in the packed red blood cells supernatant from day 7 and statistically significant data was
obtained by day 14 following storage of the red blood cells (Karon et al. 2012). Additionally,
lipid peroxidation was also found to be associated with damage of the red blood cells at the early
time points of storage and results in the subsequent release of vesicles containing cholesterol and
lipids. Studies have been carried out to determine the effects of antioxidants on the lipid
peroxidation of the red blood cells. A synthetic antioxidant called trilazad mesylate have been
found to be effective in protecting the red blood cells from damage by oxygen free radicals. The
antioxidant was found to carry out scavenging of the reactive oxygen species, thereby preventing
the occurrence of lipid peroxidation (Chadhury and Katharia 2012).
Protein oxidation
Following storage, oxidative damage results in lipid peroxidation and protein oxidation.
Increase in lipid peroxidation is associated with increase in the level of malondialdehyde.
Similarly increase in protein oxidation is associated with the increase in protein carbonylation.
Carbonylation acts as a marker of protein oxidation and increases with the onset of storage
osmotic fragility of the red blood cells and also initiates the formation of spherocytes and
echinocytes. The red blood cells with increased osmotic susceptibility are usually removed from
the circulation following transfusion (Gallagher 2013). Due to enhanced osmotic fragility, the
stored red cells undergo lysis resulting in the shedding of lipid and hemoglobin containing
vesicles in the plasma supernatant. Apart from the release of hemoglobin, enzymes like the
lactate dehydrogenase as well as potassium ions are released (Chaudhury and Katharia 2012).
Thus, these act as markers or indicators of lysis of stored red blood cells. Apart from
malondialdehyde, another compound that was found to be a suitable indicator of lipid
peroxidation was found to be 8-isoprostane. Increase in the levels of 8-isoprostane was observed
in the packed red blood cells supernatant from day 7 and statistically significant data was
obtained by day 14 following storage of the red blood cells (Karon et al. 2012). Additionally,
lipid peroxidation was also found to be associated with damage of the red blood cells at the early
time points of storage and results in the subsequent release of vesicles containing cholesterol and
lipids. Studies have been carried out to determine the effects of antioxidants on the lipid
peroxidation of the red blood cells. A synthetic antioxidant called trilazad mesylate have been
found to be effective in protecting the red blood cells from damage by oxygen free radicals. The
antioxidant was found to carry out scavenging of the reactive oxygen species, thereby preventing
the occurrence of lipid peroxidation (Chadhury and Katharia 2012).
Protein oxidation
Following storage, oxidative damage results in lipid peroxidation and protein oxidation.
Increase in lipid peroxidation is associated with increase in the level of malondialdehyde.
Similarly increase in protein oxidation is associated with the increase in protein carbonylation.
Carbonylation acts as a marker of protein oxidation and increases with the onset of storage
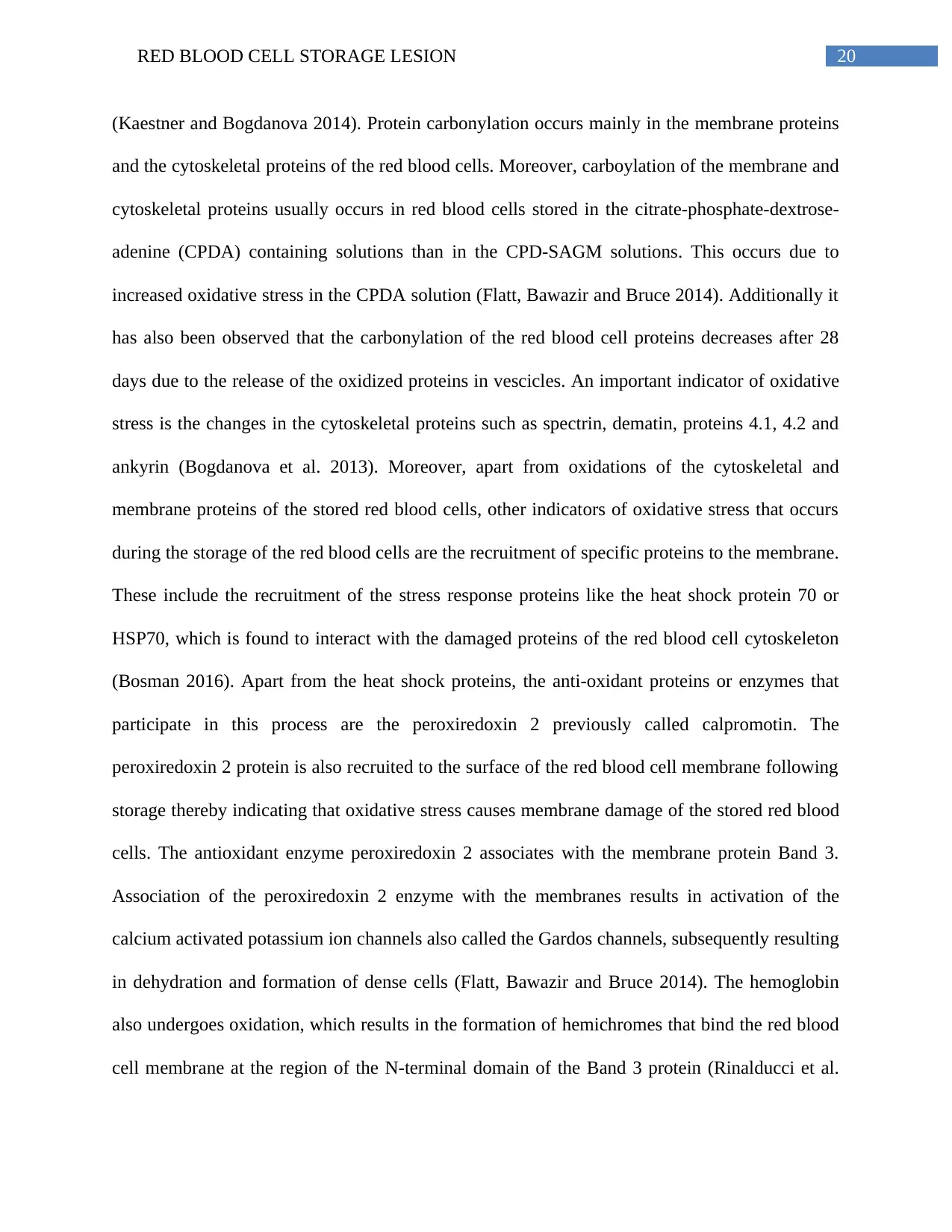
20RED BLOOD CELL STORAGE LESION
(Kaestner and Bogdanova 2014). Protein carbonylation occurs mainly in the membrane proteins
and the cytoskeletal proteins of the red blood cells. Moreover, carboylation of the membrane and
cytoskeletal proteins usually occurs in red blood cells stored in the citrate-phosphate-dextrose-
adenine (CPDA) containing solutions than in the CPD-SAGM solutions. This occurs due to
increased oxidative stress in the CPDA solution (Flatt, Bawazir and Bruce 2014). Additionally it
has also been observed that the carbonylation of the red blood cell proteins decreases after 28
days due to the release of the oxidized proteins in vescicles. An important indicator of oxidative
stress is the changes in the cytoskeletal proteins such as spectrin, dematin, proteins 4.1, 4.2 and
ankyrin (Bogdanova et al. 2013). Moreover, apart from oxidations of the cytoskeletal and
membrane proteins of the stored red blood cells, other indicators of oxidative stress that occurs
during the storage of the red blood cells are the recruitment of specific proteins to the membrane.
These include the recruitment of the stress response proteins like the heat shock protein 70 or
HSP70, which is found to interact with the damaged proteins of the red blood cell cytoskeleton
(Bosman 2016). Apart from the heat shock proteins, the anti-oxidant proteins or enzymes that
participate in this process are the peroxiredoxin 2 previously called calpromotin. The
peroxiredoxin 2 protein is also recruited to the surface of the red blood cell membrane following
storage thereby indicating that oxidative stress causes membrane damage of the stored red blood
cells. The antioxidant enzyme peroxiredoxin 2 associates with the membrane protein Band 3.
Association of the peroxiredoxin 2 enzyme with the membranes results in activation of the
calcium activated potassium ion channels also called the Gardos channels, subsequently resulting
in dehydration and formation of dense cells (Flatt, Bawazir and Bruce 2014). The hemoglobin
also undergoes oxidation, which results in the formation of hemichromes that bind the red blood
cell membrane at the region of the N-terminal domain of the Band 3 protein (Rinalducci et al.
(Kaestner and Bogdanova 2014). Protein carbonylation occurs mainly in the membrane proteins
and the cytoskeletal proteins of the red blood cells. Moreover, carboylation of the membrane and
cytoskeletal proteins usually occurs in red blood cells stored in the citrate-phosphate-dextrose-
adenine (CPDA) containing solutions than in the CPD-SAGM solutions. This occurs due to
increased oxidative stress in the CPDA solution (Flatt, Bawazir and Bruce 2014). Additionally it
has also been observed that the carbonylation of the red blood cell proteins decreases after 28
days due to the release of the oxidized proteins in vescicles. An important indicator of oxidative
stress is the changes in the cytoskeletal proteins such as spectrin, dematin, proteins 4.1, 4.2 and
ankyrin (Bogdanova et al. 2013). Moreover, apart from oxidations of the cytoskeletal and
membrane proteins of the stored red blood cells, other indicators of oxidative stress that occurs
during the storage of the red blood cells are the recruitment of specific proteins to the membrane.
These include the recruitment of the stress response proteins like the heat shock protein 70 or
HSP70, which is found to interact with the damaged proteins of the red blood cell cytoskeleton
(Bosman 2016). Apart from the heat shock proteins, the anti-oxidant proteins or enzymes that
participate in this process are the peroxiredoxin 2 previously called calpromotin. The
peroxiredoxin 2 protein is also recruited to the surface of the red blood cell membrane following
storage thereby indicating that oxidative stress causes membrane damage of the stored red blood
cells. The antioxidant enzyme peroxiredoxin 2 associates with the membrane protein Band 3.
Association of the peroxiredoxin 2 enzyme with the membranes results in activation of the
calcium activated potassium ion channels also called the Gardos channels, subsequently resulting
in dehydration and formation of dense cells (Flatt, Bawazir and Bruce 2014). The hemoglobin
also undergoes oxidation, which results in the formation of hemichromes that bind the red blood
cell membrane at the region of the N-terminal domain of the Band 3 protein (Rinalducci et al.
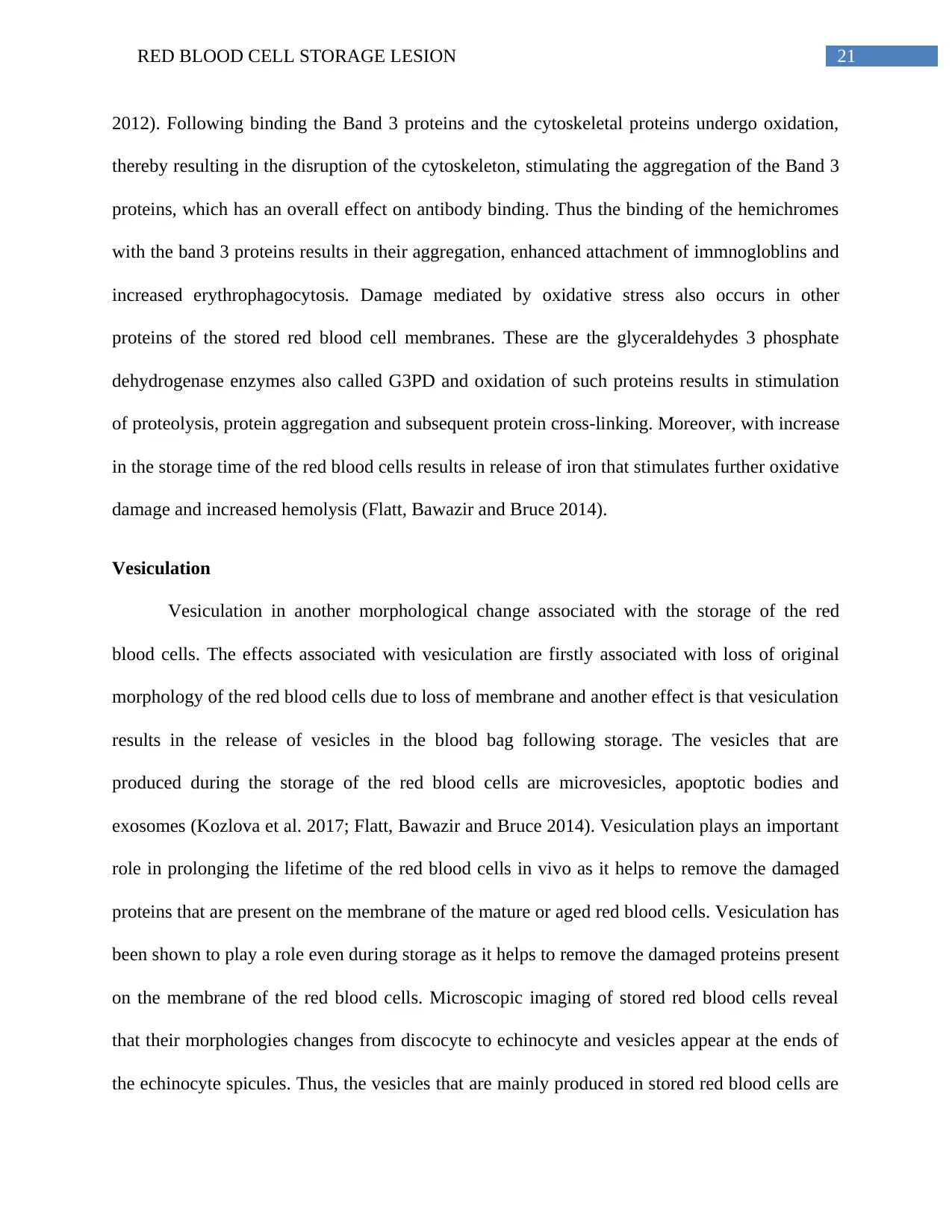
21RED BLOOD CELL STORAGE LESION
2012). Following binding the Band 3 proteins and the cytoskeletal proteins undergo oxidation,
thereby resulting in the disruption of the cytoskeleton, stimulating the aggregation of the Band 3
proteins, which has an overall effect on antibody binding. Thus the binding of the hemichromes
with the band 3 proteins results in their aggregation, enhanced attachment of immnogloblins and
increased erythrophagocytosis. Damage mediated by oxidative stress also occurs in other
proteins of the stored red blood cell membranes. These are the glyceraldehydes 3 phosphate
dehydrogenase enzymes also called G3PD and oxidation of such proteins results in stimulation
of proteolysis, protein aggregation and subsequent protein cross-linking. Moreover, with increase
in the storage time of the red blood cells results in release of iron that stimulates further oxidative
damage and increased hemolysis (Flatt, Bawazir and Bruce 2014).
Vesiculation
Vesiculation in another morphological change associated with the storage of the red
blood cells. The effects associated with vesiculation are firstly associated with loss of original
morphology of the red blood cells due to loss of membrane and another effect is that vesiculation
results in the release of vesicles in the blood bag following storage. The vesicles that are
produced during the storage of the red blood cells are microvesicles, apoptotic bodies and
exosomes (Kozlova et al. 2017; Flatt, Bawazir and Bruce 2014). Vesiculation plays an important
role in prolonging the lifetime of the red blood cells in vivo as it helps to remove the damaged
proteins that are present on the membrane of the mature or aged red blood cells. Vesiculation has
been shown to play a role even during storage as it helps to remove the damaged proteins present
on the membrane of the red blood cells. Microscopic imaging of stored red blood cells reveal
that their morphologies changes from discocyte to echinocyte and vesicles appear at the ends of
the echinocyte spicules. Thus, the vesicles that are mainly produced in stored red blood cells are
2012). Following binding the Band 3 proteins and the cytoskeletal proteins undergo oxidation,
thereby resulting in the disruption of the cytoskeleton, stimulating the aggregation of the Band 3
proteins, which has an overall effect on antibody binding. Thus the binding of the hemichromes
with the band 3 proteins results in their aggregation, enhanced attachment of immnogloblins and
increased erythrophagocytosis. Damage mediated by oxidative stress also occurs in other
proteins of the stored red blood cell membranes. These are the glyceraldehydes 3 phosphate
dehydrogenase enzymes also called G3PD and oxidation of such proteins results in stimulation
of proteolysis, protein aggregation and subsequent protein cross-linking. Moreover, with increase
in the storage time of the red blood cells results in release of iron that stimulates further oxidative
damage and increased hemolysis (Flatt, Bawazir and Bruce 2014).
Vesiculation
Vesiculation in another morphological change associated with the storage of the red
blood cells. The effects associated with vesiculation are firstly associated with loss of original
morphology of the red blood cells due to loss of membrane and another effect is that vesiculation
results in the release of vesicles in the blood bag following storage. The vesicles that are
produced during the storage of the red blood cells are microvesicles, apoptotic bodies and
exosomes (Kozlova et al. 2017; Flatt, Bawazir and Bruce 2014). Vesiculation plays an important
role in prolonging the lifetime of the red blood cells in vivo as it helps to remove the damaged
proteins that are present on the membrane of the mature or aged red blood cells. Vesiculation has
been shown to play a role even during storage as it helps to remove the damaged proteins present
on the membrane of the red blood cells. Microscopic imaging of stored red blood cells reveal
that their morphologies changes from discocyte to echinocyte and vesicles appear at the ends of
the echinocyte spicules. Thus, the vesicles that are mainly produced in stored red blood cells are
Secure Best Marks with AI Grader
Need help grading? Try our AI Grader for instant feedback on your assignments.
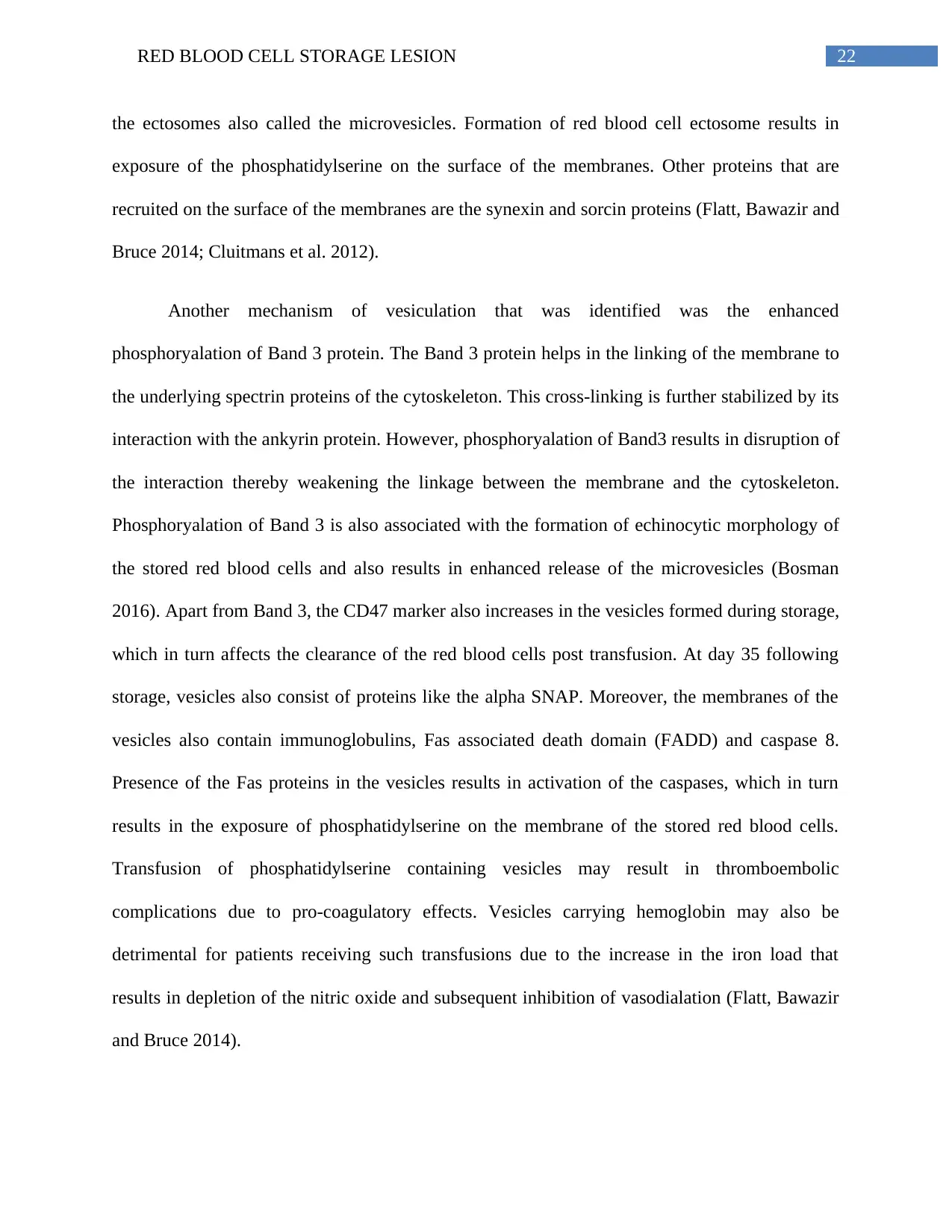
22RED BLOOD CELL STORAGE LESION
the ectosomes also called the microvesicles. Formation of red blood cell ectosome results in
exposure of the phosphatidylserine on the surface of the membranes. Other proteins that are
recruited on the surface of the membranes are the synexin and sorcin proteins (Flatt, Bawazir and
Bruce 2014; Cluitmans et al. 2012).
Another mechanism of vesiculation that was identified was the enhanced
phosphoryalation of Band 3 protein. The Band 3 protein helps in the linking of the membrane to
the underlying spectrin proteins of the cytoskeleton. This cross-linking is further stabilized by its
interaction with the ankyrin protein. However, phosphoryalation of Band3 results in disruption of
the interaction thereby weakening the linkage between the membrane and the cytoskeleton.
Phosphoryalation of Band 3 is also associated with the formation of echinocytic morphology of
the stored red blood cells and also results in enhanced release of the microvesicles (Bosman
2016). Apart from Band 3, the CD47 marker also increases in the vesicles formed during storage,
which in turn affects the clearance of the red blood cells post transfusion. At day 35 following
storage, vesicles also consist of proteins like the alpha SNAP. Moreover, the membranes of the
vesicles also contain immunoglobulins, Fas associated death domain (FADD) and caspase 8.
Presence of the Fas proteins in the vesicles results in activation of the caspases, which in turn
results in the exposure of phosphatidylserine on the membrane of the stored red blood cells.
Transfusion of phosphatidylserine containing vesicles may result in thromboembolic
complications due to pro-coagulatory effects. Vesicles carrying hemoglobin may also be
detrimental for patients receiving such transfusions due to the increase in the iron load that
results in depletion of the nitric oxide and subsequent inhibition of vasodialation (Flatt, Bawazir
and Bruce 2014).
the ectosomes also called the microvesicles. Formation of red blood cell ectosome results in
exposure of the phosphatidylserine on the surface of the membranes. Other proteins that are
recruited on the surface of the membranes are the synexin and sorcin proteins (Flatt, Bawazir and
Bruce 2014; Cluitmans et al. 2012).
Another mechanism of vesiculation that was identified was the enhanced
phosphoryalation of Band 3 protein. The Band 3 protein helps in the linking of the membrane to
the underlying spectrin proteins of the cytoskeleton. This cross-linking is further stabilized by its
interaction with the ankyrin protein. However, phosphoryalation of Band3 results in disruption of
the interaction thereby weakening the linkage between the membrane and the cytoskeleton.
Phosphoryalation of Band 3 is also associated with the formation of echinocytic morphology of
the stored red blood cells and also results in enhanced release of the microvesicles (Bosman
2016). Apart from Band 3, the CD47 marker also increases in the vesicles formed during storage,
which in turn affects the clearance of the red blood cells post transfusion. At day 35 following
storage, vesicles also consist of proteins like the alpha SNAP. Moreover, the membranes of the
vesicles also contain immunoglobulins, Fas associated death domain (FADD) and caspase 8.
Presence of the Fas proteins in the vesicles results in activation of the caspases, which in turn
results in the exposure of phosphatidylserine on the membrane of the stored red blood cells.
Transfusion of phosphatidylserine containing vesicles may result in thromboembolic
complications due to pro-coagulatory effects. Vesicles carrying hemoglobin may also be
detrimental for patients receiving such transfusions due to the increase in the iron load that
results in depletion of the nitric oxide and subsequent inhibition of vasodialation (Flatt, Bawazir
and Bruce 2014).
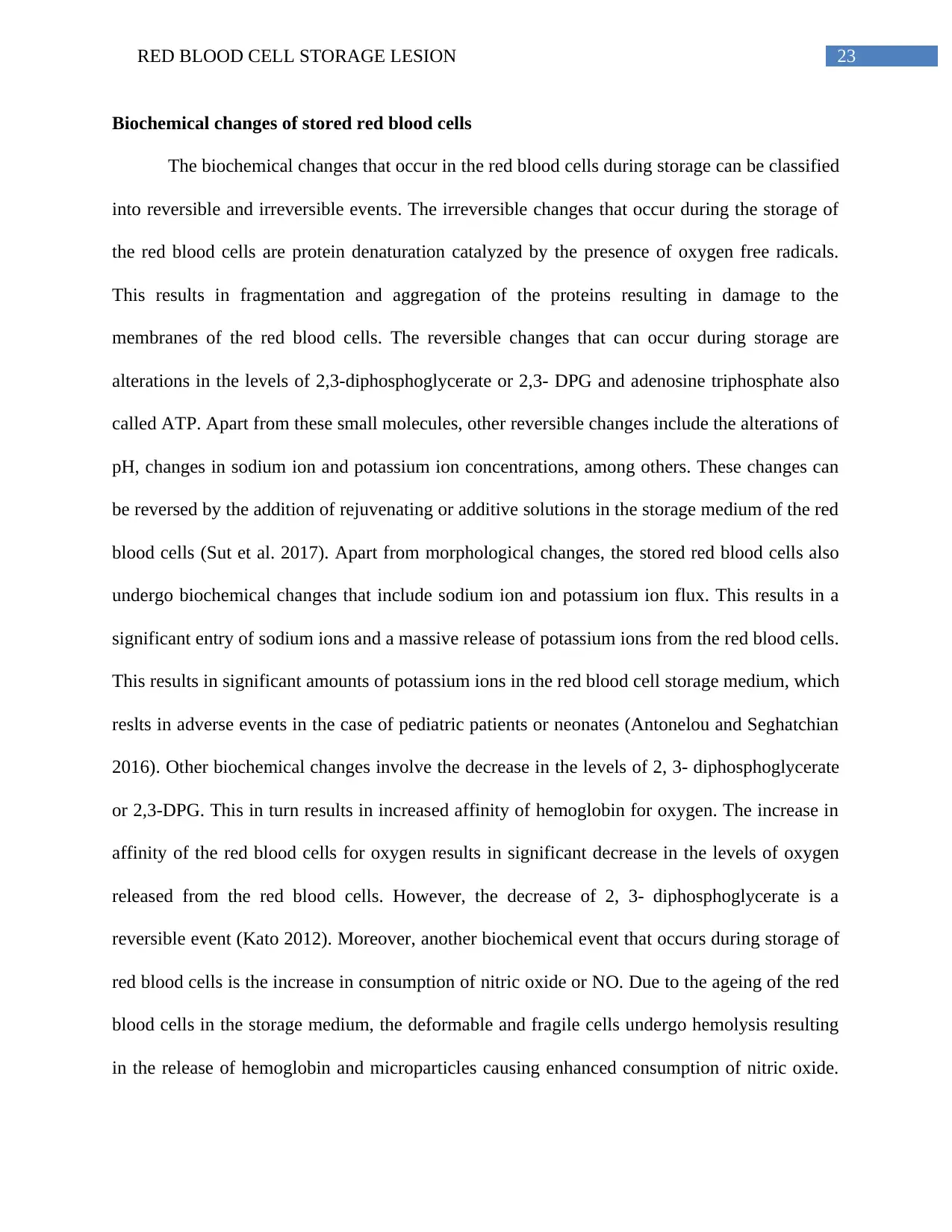
23RED BLOOD CELL STORAGE LESION
Biochemical changes of stored red blood cells
The biochemical changes that occur in the red blood cells during storage can be classified
into reversible and irreversible events. The irreversible changes that occur during the storage of
the red blood cells are protein denaturation catalyzed by the presence of oxygen free radicals.
This results in fragmentation and aggregation of the proteins resulting in damage to the
membranes of the red blood cells. The reversible changes that can occur during storage are
alterations in the levels of 2,3-diphosphoglycerate or 2,3- DPG and adenosine triphosphate also
called ATP. Apart from these small molecules, other reversible changes include the alterations of
pH, changes in sodium ion and potassium ion concentrations, among others. These changes can
be reversed by the addition of rejuvenating or additive solutions in the storage medium of the red
blood cells (Sut et al. 2017). Apart from morphological changes, the stored red blood cells also
undergo biochemical changes that include sodium ion and potassium ion flux. This results in a
significant entry of sodium ions and a massive release of potassium ions from the red blood cells.
This results in significant amounts of potassium ions in the red blood cell storage medium, which
reslts in adverse events in the case of pediatric patients or neonates (Antonelou and Seghatchian
2016). Other biochemical changes involve the decrease in the levels of 2, 3- diphosphoglycerate
or 2,3-DPG. This in turn results in increased affinity of hemoglobin for oxygen. The increase in
affinity of the red blood cells for oxygen results in significant decrease in the levels of oxygen
released from the red blood cells. However, the decrease of 2, 3- diphosphoglycerate is a
reversible event (Kato 2012). Moreover, another biochemical event that occurs during storage of
red blood cells is the increase in consumption of nitric oxide or NO. Due to the ageing of the red
blood cells in the storage medium, the deformable and fragile cells undergo hemolysis resulting
in the release of hemoglobin and microparticles causing enhanced consumption of nitric oxide.
Biochemical changes of stored red blood cells
The biochemical changes that occur in the red blood cells during storage can be classified
into reversible and irreversible events. The irreversible changes that occur during the storage of
the red blood cells are protein denaturation catalyzed by the presence of oxygen free radicals.
This results in fragmentation and aggregation of the proteins resulting in damage to the
membranes of the red blood cells. The reversible changes that can occur during storage are
alterations in the levels of 2,3-diphosphoglycerate or 2,3- DPG and adenosine triphosphate also
called ATP. Apart from these small molecules, other reversible changes include the alterations of
pH, changes in sodium ion and potassium ion concentrations, among others. These changes can
be reversed by the addition of rejuvenating or additive solutions in the storage medium of the red
blood cells (Sut et al. 2017). Apart from morphological changes, the stored red blood cells also
undergo biochemical changes that include sodium ion and potassium ion flux. This results in a
significant entry of sodium ions and a massive release of potassium ions from the red blood cells.
This results in significant amounts of potassium ions in the red blood cell storage medium, which
reslts in adverse events in the case of pediatric patients or neonates (Antonelou and Seghatchian
2016). Other biochemical changes involve the decrease in the levels of 2, 3- diphosphoglycerate
or 2,3-DPG. This in turn results in increased affinity of hemoglobin for oxygen. The increase in
affinity of the red blood cells for oxygen results in significant decrease in the levels of oxygen
released from the red blood cells. However, the decrease of 2, 3- diphosphoglycerate is a
reversible event (Kato 2012). Moreover, another biochemical event that occurs during storage of
red blood cells is the increase in consumption of nitric oxide or NO. Due to the ageing of the red
blood cells in the storage medium, the deformable and fragile cells undergo hemolysis resulting
in the release of hemoglobin and microparticles causing enhanced consumption of nitric oxide.
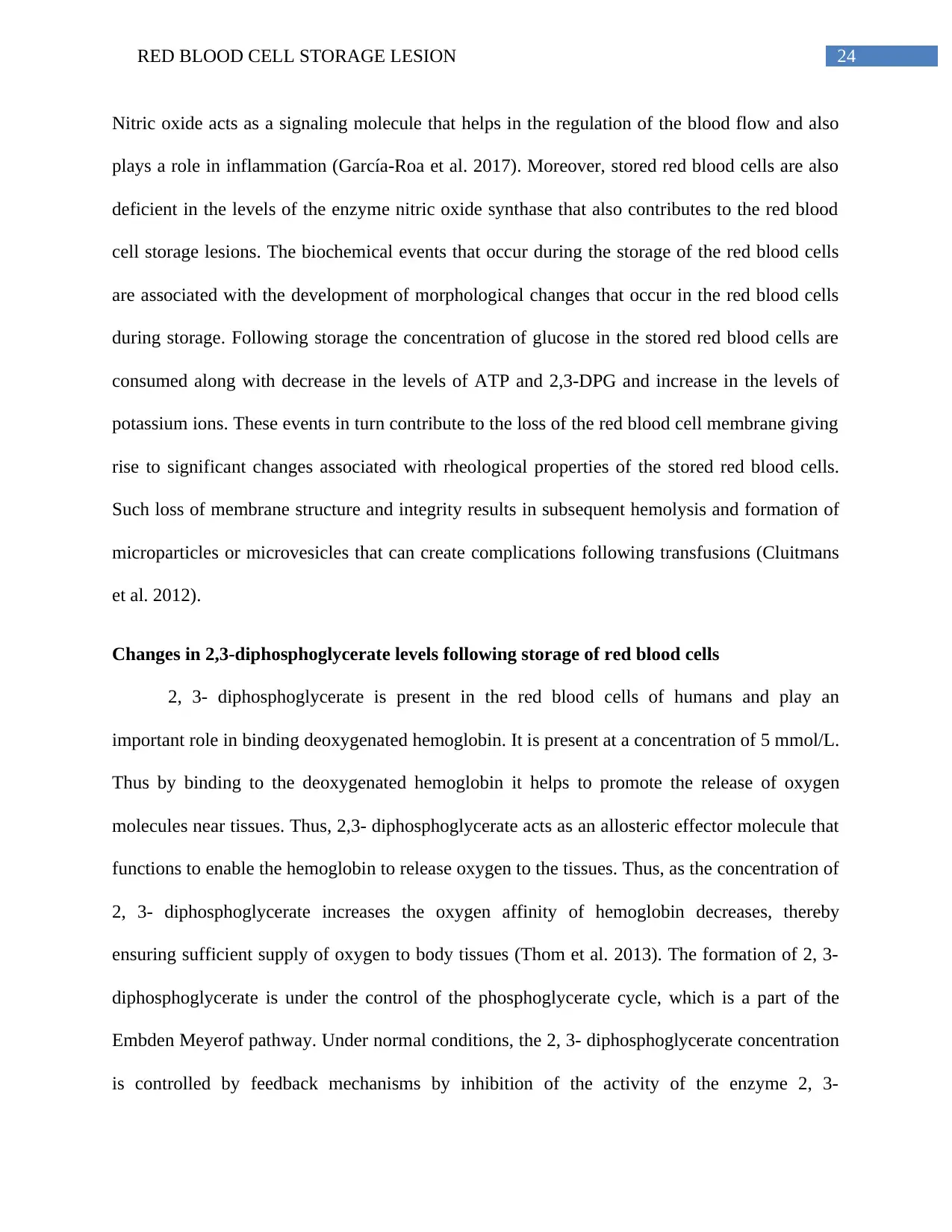
24RED BLOOD CELL STORAGE LESION
Nitric oxide acts as a signaling molecule that helps in the regulation of the blood flow and also
plays a role in inflammation (García-Roa et al. 2017). Moreover, stored red blood cells are also
deficient in the levels of the enzyme nitric oxide synthase that also contributes to the red blood
cell storage lesions. The biochemical events that occur during the storage of the red blood cells
are associated with the development of morphological changes that occur in the red blood cells
during storage. Following storage the concentration of glucose in the stored red blood cells are
consumed along with decrease in the levels of ATP and 2,3-DPG and increase in the levels of
potassium ions. These events in turn contribute to the loss of the red blood cell membrane giving
rise to significant changes associated with rheological properties of the stored red blood cells.
Such loss of membrane structure and integrity results in subsequent hemolysis and formation of
microparticles or microvesicles that can create complications following transfusions (Cluitmans
et al. 2012).
Changes in 2,3-diphosphoglycerate levels following storage of red blood cells
2, 3- diphosphoglycerate is present in the red blood cells of humans and play an
important role in binding deoxygenated hemoglobin. It is present at a concentration of 5 mmol/L.
Thus by binding to the deoxygenated hemoglobin it helps to promote the release of oxygen
molecules near tissues. Thus, 2,3- diphosphoglycerate acts as an allosteric effector molecule that
functions to enable the hemoglobin to release oxygen to the tissues. Thus, as the concentration of
2, 3- diphosphoglycerate increases the oxygen affinity of hemoglobin decreases, thereby
ensuring sufficient supply of oxygen to body tissues (Thom et al. 2013). The formation of 2, 3-
diphosphoglycerate is under the control of the phosphoglycerate cycle, which is a part of the
Embden Meyerof pathway. Under normal conditions, the 2, 3- diphosphoglycerate concentration
is controlled by feedback mechanisms by inhibition of the activity of the enzyme 2, 3-
Nitric oxide acts as a signaling molecule that helps in the regulation of the blood flow and also
plays a role in inflammation (García-Roa et al. 2017). Moreover, stored red blood cells are also
deficient in the levels of the enzyme nitric oxide synthase that also contributes to the red blood
cell storage lesions. The biochemical events that occur during the storage of the red blood cells
are associated with the development of morphological changes that occur in the red blood cells
during storage. Following storage the concentration of glucose in the stored red blood cells are
consumed along with decrease in the levels of ATP and 2,3-DPG and increase in the levels of
potassium ions. These events in turn contribute to the loss of the red blood cell membrane giving
rise to significant changes associated with rheological properties of the stored red blood cells.
Such loss of membrane structure and integrity results in subsequent hemolysis and formation of
microparticles or microvesicles that can create complications following transfusions (Cluitmans
et al. 2012).
Changes in 2,3-diphosphoglycerate levels following storage of red blood cells
2, 3- diphosphoglycerate is present in the red blood cells of humans and play an
important role in binding deoxygenated hemoglobin. It is present at a concentration of 5 mmol/L.
Thus by binding to the deoxygenated hemoglobin it helps to promote the release of oxygen
molecules near tissues. Thus, 2,3- diphosphoglycerate acts as an allosteric effector molecule that
functions to enable the hemoglobin to release oxygen to the tissues. Thus, as the concentration of
2, 3- diphosphoglycerate increases the oxygen affinity of hemoglobin decreases, thereby
ensuring sufficient supply of oxygen to body tissues (Thom et al. 2013). The formation of 2, 3-
diphosphoglycerate is under the control of the phosphoglycerate cycle, which is a part of the
Embden Meyerof pathway. Under normal conditions, the 2, 3- diphosphoglycerate concentration
is controlled by feedback mechanisms by inhibition of the activity of the enzyme 2, 3-
Paraphrase This Document
Need a fresh take? Get an instant paraphrase of this document with our AI Paraphraser
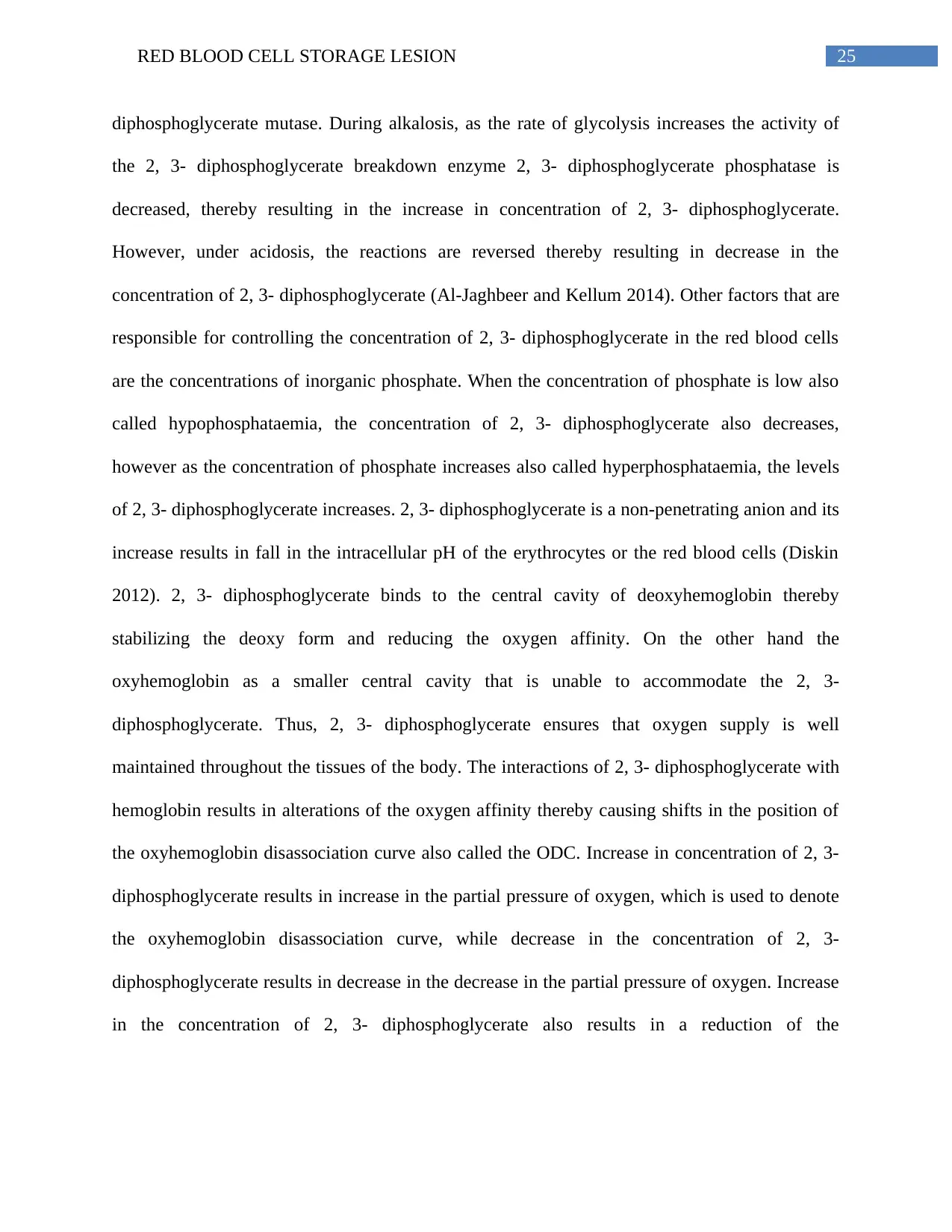
25RED BLOOD CELL STORAGE LESION
diphosphoglycerate mutase. During alkalosis, as the rate of glycolysis increases the activity of
the 2, 3- diphosphoglycerate breakdown enzyme 2, 3- diphosphoglycerate phosphatase is
decreased, thereby resulting in the increase in concentration of 2, 3- diphosphoglycerate.
However, under acidosis, the reactions are reversed thereby resulting in decrease in the
concentration of 2, 3- diphosphoglycerate (Al-Jaghbeer and Kellum 2014). Other factors that are
responsible for controlling the concentration of 2, 3- diphosphoglycerate in the red blood cells
are the concentrations of inorganic phosphate. When the concentration of phosphate is low also
called hypophosphataemia, the concentration of 2, 3- diphosphoglycerate also decreases,
however as the concentration of phosphate increases also called hyperphosphataemia, the levels
of 2, 3- diphosphoglycerate increases. 2, 3- diphosphoglycerate is a non-penetrating anion and its
increase results in fall in the intracellular pH of the erythrocytes or the red blood cells (Diskin
2012). 2, 3- diphosphoglycerate binds to the central cavity of deoxyhemoglobin thereby
stabilizing the deoxy form and reducing the oxygen affinity. On the other hand the
oxyhemoglobin as a smaller central cavity that is unable to accommodate the 2, 3-
diphosphoglycerate. Thus, 2, 3- diphosphoglycerate ensures that oxygen supply is well
maintained throughout the tissues of the body. The interactions of 2, 3- diphosphoglycerate with
hemoglobin results in alterations of the oxygen affinity thereby causing shifts in the position of
the oxyhemoglobin disassociation curve also called the ODC. Increase in concentration of 2, 3-
diphosphoglycerate results in increase in the partial pressure of oxygen, which is used to denote
the oxyhemoglobin disassociation curve, while decrease in the concentration of 2, 3-
diphosphoglycerate results in decrease in the decrease in the partial pressure of oxygen. Increase
in the concentration of 2, 3- diphosphoglycerate also results in a reduction of the
diphosphoglycerate mutase. During alkalosis, as the rate of glycolysis increases the activity of
the 2, 3- diphosphoglycerate breakdown enzyme 2, 3- diphosphoglycerate phosphatase is
decreased, thereby resulting in the increase in concentration of 2, 3- diphosphoglycerate.
However, under acidosis, the reactions are reversed thereby resulting in decrease in the
concentration of 2, 3- diphosphoglycerate (Al-Jaghbeer and Kellum 2014). Other factors that are
responsible for controlling the concentration of 2, 3- diphosphoglycerate in the red blood cells
are the concentrations of inorganic phosphate. When the concentration of phosphate is low also
called hypophosphataemia, the concentration of 2, 3- diphosphoglycerate also decreases,
however as the concentration of phosphate increases also called hyperphosphataemia, the levels
of 2, 3- diphosphoglycerate increases. 2, 3- diphosphoglycerate is a non-penetrating anion and its
increase results in fall in the intracellular pH of the erythrocytes or the red blood cells (Diskin
2012). 2, 3- diphosphoglycerate binds to the central cavity of deoxyhemoglobin thereby
stabilizing the deoxy form and reducing the oxygen affinity. On the other hand the
oxyhemoglobin as a smaller central cavity that is unable to accommodate the 2, 3-
diphosphoglycerate. Thus, 2, 3- diphosphoglycerate ensures that oxygen supply is well
maintained throughout the tissues of the body. The interactions of 2, 3- diphosphoglycerate with
hemoglobin results in alterations of the oxygen affinity thereby causing shifts in the position of
the oxyhemoglobin disassociation curve also called the ODC. Increase in concentration of 2, 3-
diphosphoglycerate results in increase in the partial pressure of oxygen, which is used to denote
the oxyhemoglobin disassociation curve, while decrease in the concentration of 2, 3-
diphosphoglycerate results in decrease in the decrease in the partial pressure of oxygen. Increase
in the concentration of 2, 3- diphosphoglycerate also results in a reduction of the
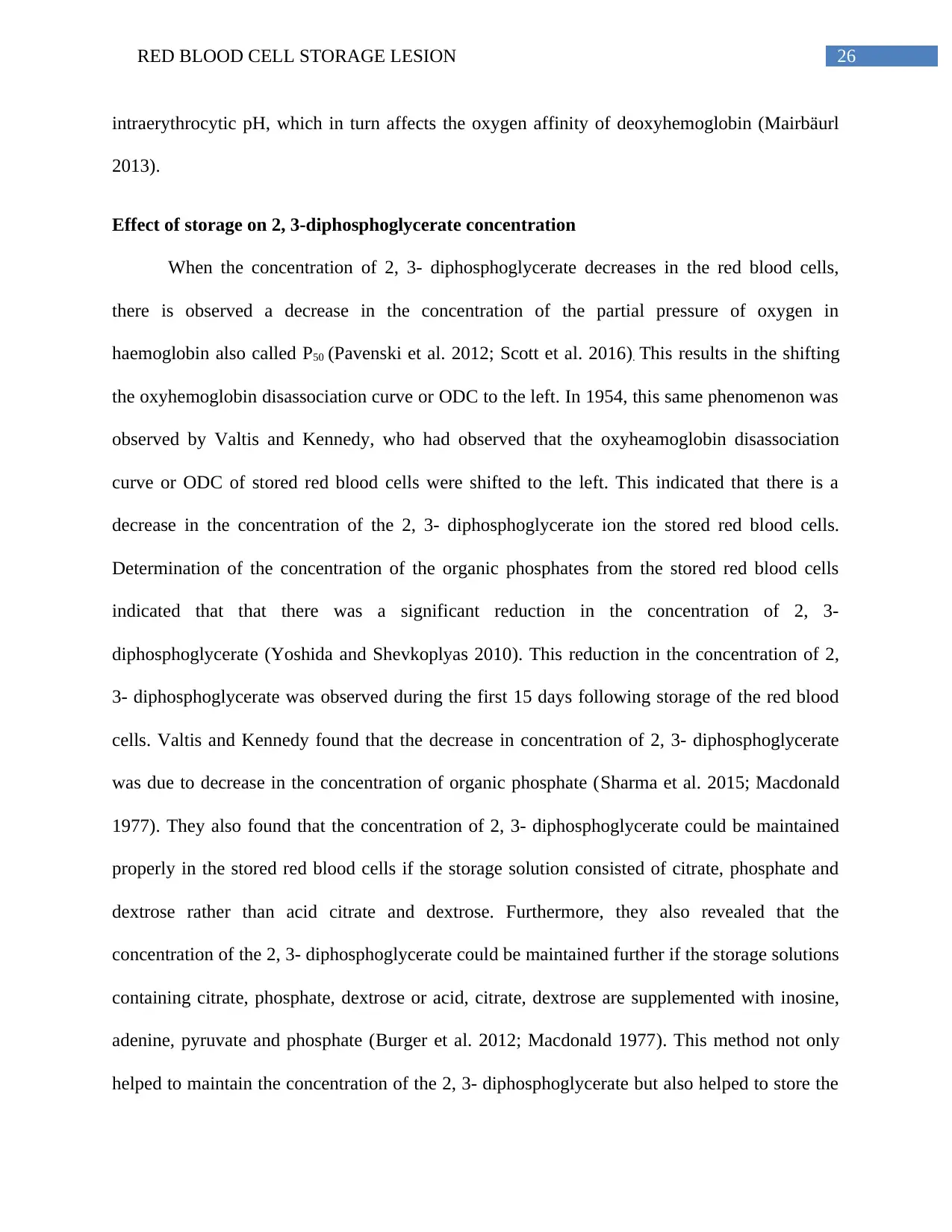
26RED BLOOD CELL STORAGE LESION
intraerythrocytic pH, which in turn affects the oxygen affinity of deoxyhemoglobin (Mairbäurl
2013).
Effect of storage on 2, 3-diphosphoglycerate concentration
When the concentration of 2, 3- diphosphoglycerate decreases in the red blood cells,
there is observed a decrease in the concentration of the partial pressure of oxygen in
haemoglobin also called P50 (Pavenski et al. 2012; Scott et al. 2016). This results in the shifting
the oxyhemoglobin disassociation curve or ODC to the left. In 1954, this same phenomenon was
observed by Valtis and Kennedy, who had observed that the oxyheamoglobin disassociation
curve or ODC of stored red blood cells were shifted to the left. This indicated that there is a
decrease in the concentration of the 2, 3- diphosphoglycerate ion the stored red blood cells.
Determination of the concentration of the organic phosphates from the stored red blood cells
indicated that that there was a significant reduction in the concentration of 2, 3-
diphosphoglycerate (Yoshida and Shevkoplyas 2010). This reduction in the concentration of 2,
3- diphosphoglycerate was observed during the first 15 days following storage of the red blood
cells. Valtis and Kennedy found that the decrease in concentration of 2, 3- diphosphoglycerate
was due to decrease in the concentration of organic phosphate (Sharma et al. 2015; Macdonald
1977). They also found that the concentration of 2, 3- diphosphoglycerate could be maintained
properly in the stored red blood cells if the storage solution consisted of citrate, phosphate and
dextrose rather than acid citrate and dextrose. Furthermore, they also revealed that the
concentration of the 2, 3- diphosphoglycerate could be maintained further if the storage solutions
containing citrate, phosphate, dextrose or acid, citrate, dextrose are supplemented with inosine,
adenine, pyruvate and phosphate (Burger et al. 2012; Macdonald 1977). This method not only
helped to maintain the concentration of the 2, 3- diphosphoglycerate but also helped to store the
intraerythrocytic pH, which in turn affects the oxygen affinity of deoxyhemoglobin (Mairbäurl
2013).
Effect of storage on 2, 3-diphosphoglycerate concentration
When the concentration of 2, 3- diphosphoglycerate decreases in the red blood cells,
there is observed a decrease in the concentration of the partial pressure of oxygen in
haemoglobin also called P50 (Pavenski et al. 2012; Scott et al. 2016). This results in the shifting
the oxyhemoglobin disassociation curve or ODC to the left. In 1954, this same phenomenon was
observed by Valtis and Kennedy, who had observed that the oxyheamoglobin disassociation
curve or ODC of stored red blood cells were shifted to the left. This indicated that there is a
decrease in the concentration of the 2, 3- diphosphoglycerate ion the stored red blood cells.
Determination of the concentration of the organic phosphates from the stored red blood cells
indicated that that there was a significant reduction in the concentration of 2, 3-
diphosphoglycerate (Yoshida and Shevkoplyas 2010). This reduction in the concentration of 2,
3- diphosphoglycerate was observed during the first 15 days following storage of the red blood
cells. Valtis and Kennedy found that the decrease in concentration of 2, 3- diphosphoglycerate
was due to decrease in the concentration of organic phosphate (Sharma et al. 2015; Macdonald
1977). They also found that the concentration of 2, 3- diphosphoglycerate could be maintained
properly in the stored red blood cells if the storage solution consisted of citrate, phosphate and
dextrose rather than acid citrate and dextrose. Furthermore, they also revealed that the
concentration of the 2, 3- diphosphoglycerate could be maintained further if the storage solutions
containing citrate, phosphate, dextrose or acid, citrate, dextrose are supplemented with inosine,
adenine, pyruvate and phosphate (Burger et al. 2012; Macdonald 1977). This method not only
helped to maintain the concentration of the 2, 3- diphosphoglycerate but also helped to store the
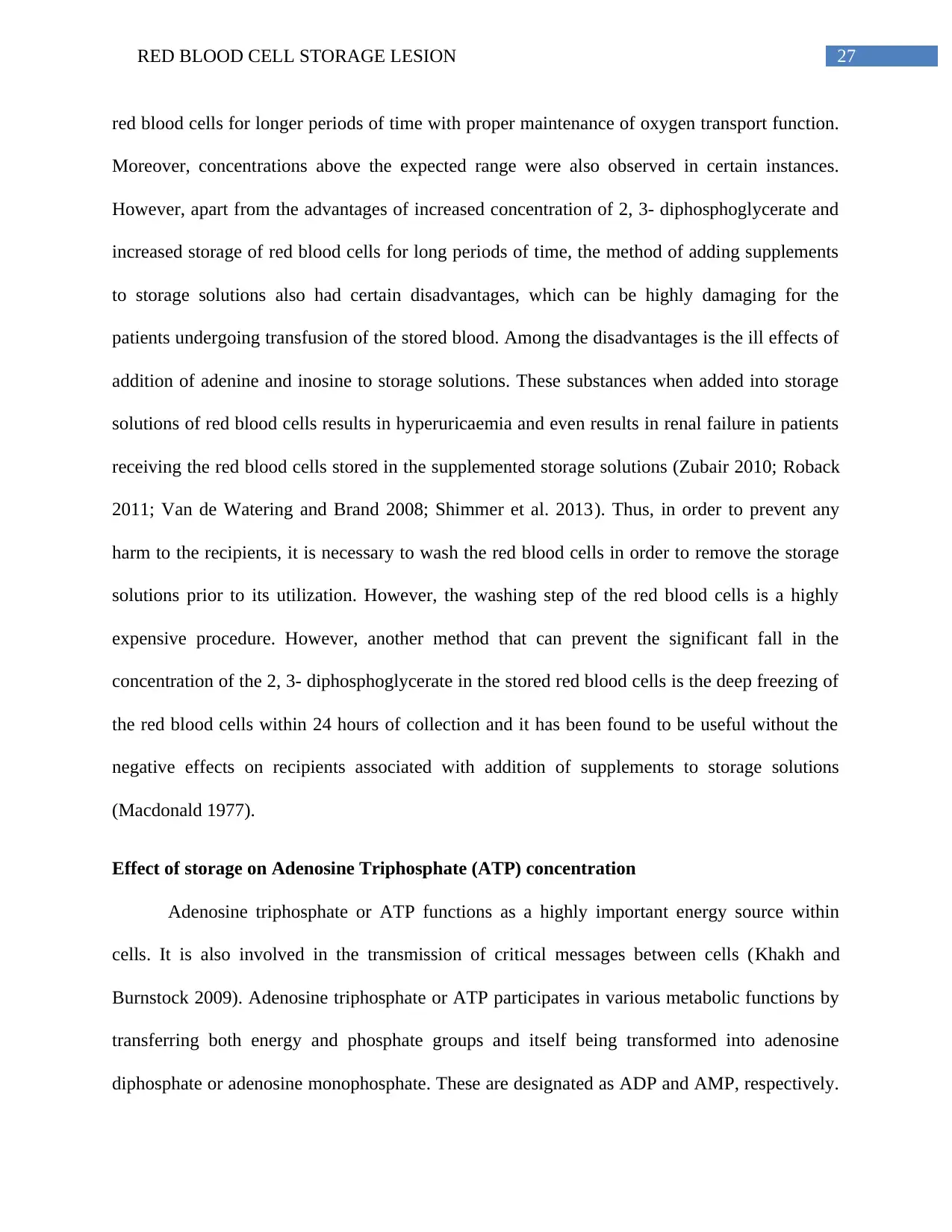
27RED BLOOD CELL STORAGE LESION
red blood cells for longer periods of time with proper maintenance of oxygen transport function.
Moreover, concentrations above the expected range were also observed in certain instances.
However, apart from the advantages of increased concentration of 2, 3- diphosphoglycerate and
increased storage of red blood cells for long periods of time, the method of adding supplements
to storage solutions also had certain disadvantages, which can be highly damaging for the
patients undergoing transfusion of the stored blood. Among the disadvantages is the ill effects of
addition of adenine and inosine to storage solutions. These substances when added into storage
solutions of red blood cells results in hyperuricaemia and even results in renal failure in patients
receiving the red blood cells stored in the supplemented storage solutions (Zubair 2010; Roback
2011; Van de Watering and Brand 2008; Shimmer et al. 2013). Thus, in order to prevent any
harm to the recipients, it is necessary to wash the red blood cells in order to remove the storage
solutions prior to its utilization. However, the washing step of the red blood cells is a highly
expensive procedure. However, another method that can prevent the significant fall in the
concentration of the 2, 3- diphosphoglycerate in the stored red blood cells is the deep freezing of
the red blood cells within 24 hours of collection and it has been found to be useful without the
negative effects on recipients associated with addition of supplements to storage solutions
(Macdonald 1977).
Effect of storage on Adenosine Triphosphate (ATP) concentration
Adenosine triphosphate or ATP functions as a highly important energy source within
cells. It is also involved in the transmission of critical messages between cells (Khakh and
Burnstock 2009). Adenosine triphosphate or ATP participates in various metabolic functions by
transferring both energy and phosphate groups and itself being transformed into adenosine
diphosphate or adenosine monophosphate. These are designated as ADP and AMP, respectively.
red blood cells for longer periods of time with proper maintenance of oxygen transport function.
Moreover, concentrations above the expected range were also observed in certain instances.
However, apart from the advantages of increased concentration of 2, 3- diphosphoglycerate and
increased storage of red blood cells for long periods of time, the method of adding supplements
to storage solutions also had certain disadvantages, which can be highly damaging for the
patients undergoing transfusion of the stored blood. Among the disadvantages is the ill effects of
addition of adenine and inosine to storage solutions. These substances when added into storage
solutions of red blood cells results in hyperuricaemia and even results in renal failure in patients
receiving the red blood cells stored in the supplemented storage solutions (Zubair 2010; Roback
2011; Van de Watering and Brand 2008; Shimmer et al. 2013). Thus, in order to prevent any
harm to the recipients, it is necessary to wash the red blood cells in order to remove the storage
solutions prior to its utilization. However, the washing step of the red blood cells is a highly
expensive procedure. However, another method that can prevent the significant fall in the
concentration of the 2, 3- diphosphoglycerate in the stored red blood cells is the deep freezing of
the red blood cells within 24 hours of collection and it has been found to be useful without the
negative effects on recipients associated with addition of supplements to storage solutions
(Macdonald 1977).
Effect of storage on Adenosine Triphosphate (ATP) concentration
Adenosine triphosphate or ATP functions as a highly important energy source within
cells. It is also involved in the transmission of critical messages between cells (Khakh and
Burnstock 2009). Adenosine triphosphate or ATP participates in various metabolic functions by
transferring both energy and phosphate groups and itself being transformed into adenosine
diphosphate or adenosine monophosphate. These are designated as ADP and AMP, respectively.
Secure Best Marks with AI Grader
Need help grading? Try our AI Grader for instant feedback on your assignments.
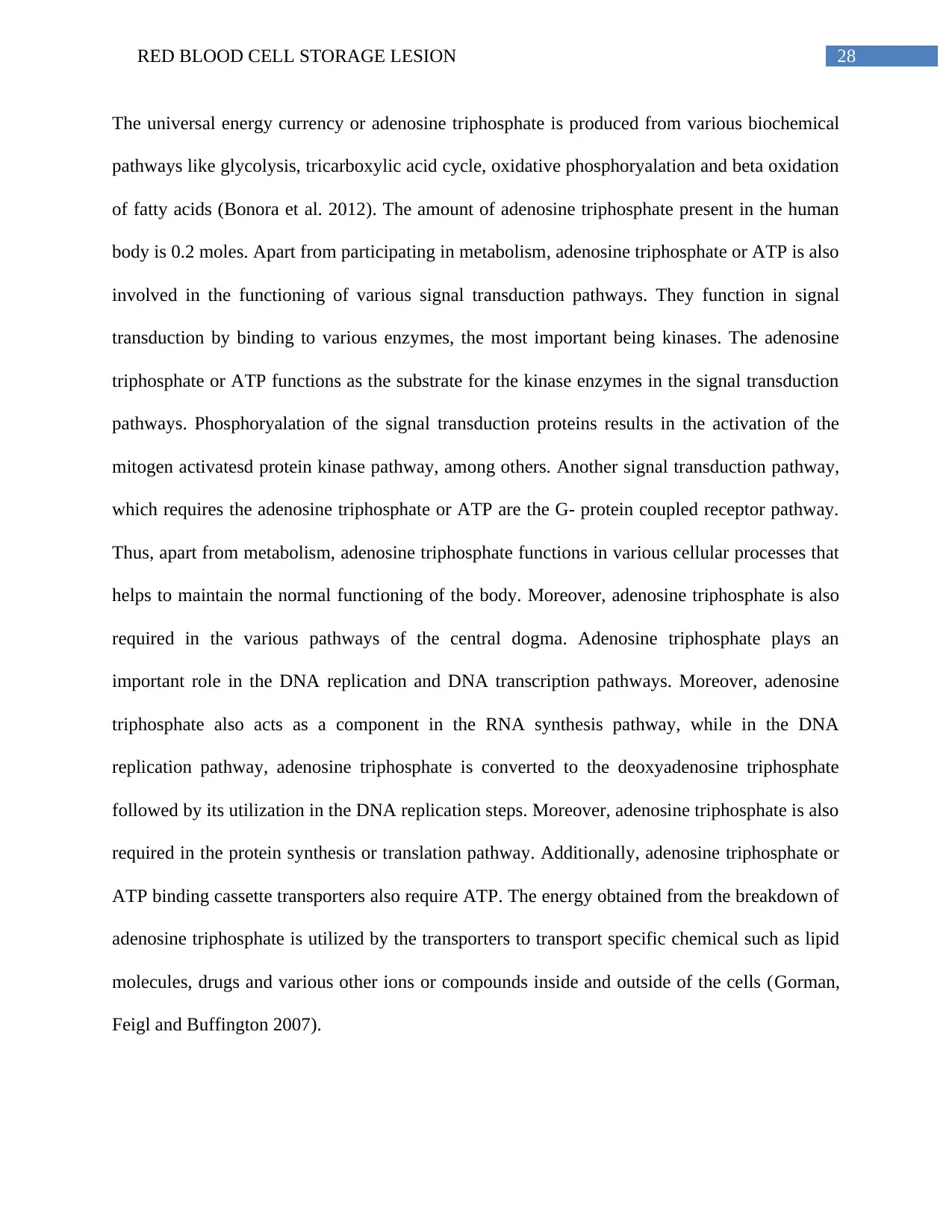
28RED BLOOD CELL STORAGE LESION
The universal energy currency or adenosine triphosphate is produced from various biochemical
pathways like glycolysis, tricarboxylic acid cycle, oxidative phosphoryalation and beta oxidation
of fatty acids (Bonora et al. 2012). The amount of adenosine triphosphate present in the human
body is 0.2 moles. Apart from participating in metabolism, adenosine triphosphate or ATP is also
involved in the functioning of various signal transduction pathways. They function in signal
transduction by binding to various enzymes, the most important being kinases. The adenosine
triphosphate or ATP functions as the substrate for the kinase enzymes in the signal transduction
pathways. Phosphoryalation of the signal transduction proteins results in the activation of the
mitogen activatesd protein kinase pathway, among others. Another signal transduction pathway,
which requires the adenosine triphosphate or ATP are the G- protein coupled receptor pathway.
Thus, apart from metabolism, adenosine triphosphate functions in various cellular processes that
helps to maintain the normal functioning of the body. Moreover, adenosine triphosphate is also
required in the various pathways of the central dogma. Adenosine triphosphate plays an
important role in the DNA replication and DNA transcription pathways. Moreover, adenosine
triphosphate also acts as a component in the RNA synthesis pathway, while in the DNA
replication pathway, adenosine triphosphate is converted to the deoxyadenosine triphosphate
followed by its utilization in the DNA replication steps. Moreover, adenosine triphosphate is also
required in the protein synthesis or translation pathway. Additionally, adenosine triphosphate or
ATP binding cassette transporters also require ATP. The energy obtained from the breakdown of
adenosine triphosphate is utilized by the transporters to transport specific chemical such as lipid
molecules, drugs and various other ions or compounds inside and outside of the cells (Gorman,
Feigl and Buffington 2007).
The universal energy currency or adenosine triphosphate is produced from various biochemical
pathways like glycolysis, tricarboxylic acid cycle, oxidative phosphoryalation and beta oxidation
of fatty acids (Bonora et al. 2012). The amount of adenosine triphosphate present in the human
body is 0.2 moles. Apart from participating in metabolism, adenosine triphosphate or ATP is also
involved in the functioning of various signal transduction pathways. They function in signal
transduction by binding to various enzymes, the most important being kinases. The adenosine
triphosphate or ATP functions as the substrate for the kinase enzymes in the signal transduction
pathways. Phosphoryalation of the signal transduction proteins results in the activation of the
mitogen activatesd protein kinase pathway, among others. Another signal transduction pathway,
which requires the adenosine triphosphate or ATP are the G- protein coupled receptor pathway.
Thus, apart from metabolism, adenosine triphosphate functions in various cellular processes that
helps to maintain the normal functioning of the body. Moreover, adenosine triphosphate is also
required in the various pathways of the central dogma. Adenosine triphosphate plays an
important role in the DNA replication and DNA transcription pathways. Moreover, adenosine
triphosphate also acts as a component in the RNA synthesis pathway, while in the DNA
replication pathway, adenosine triphosphate is converted to the deoxyadenosine triphosphate
followed by its utilization in the DNA replication steps. Moreover, adenosine triphosphate is also
required in the protein synthesis or translation pathway. Additionally, adenosine triphosphate or
ATP binding cassette transporters also require ATP. The energy obtained from the breakdown of
adenosine triphosphate is utilized by the transporters to transport specific chemical such as lipid
molecules, drugs and various other ions or compounds inside and outside of the cells (Gorman,
Feigl and Buffington 2007).
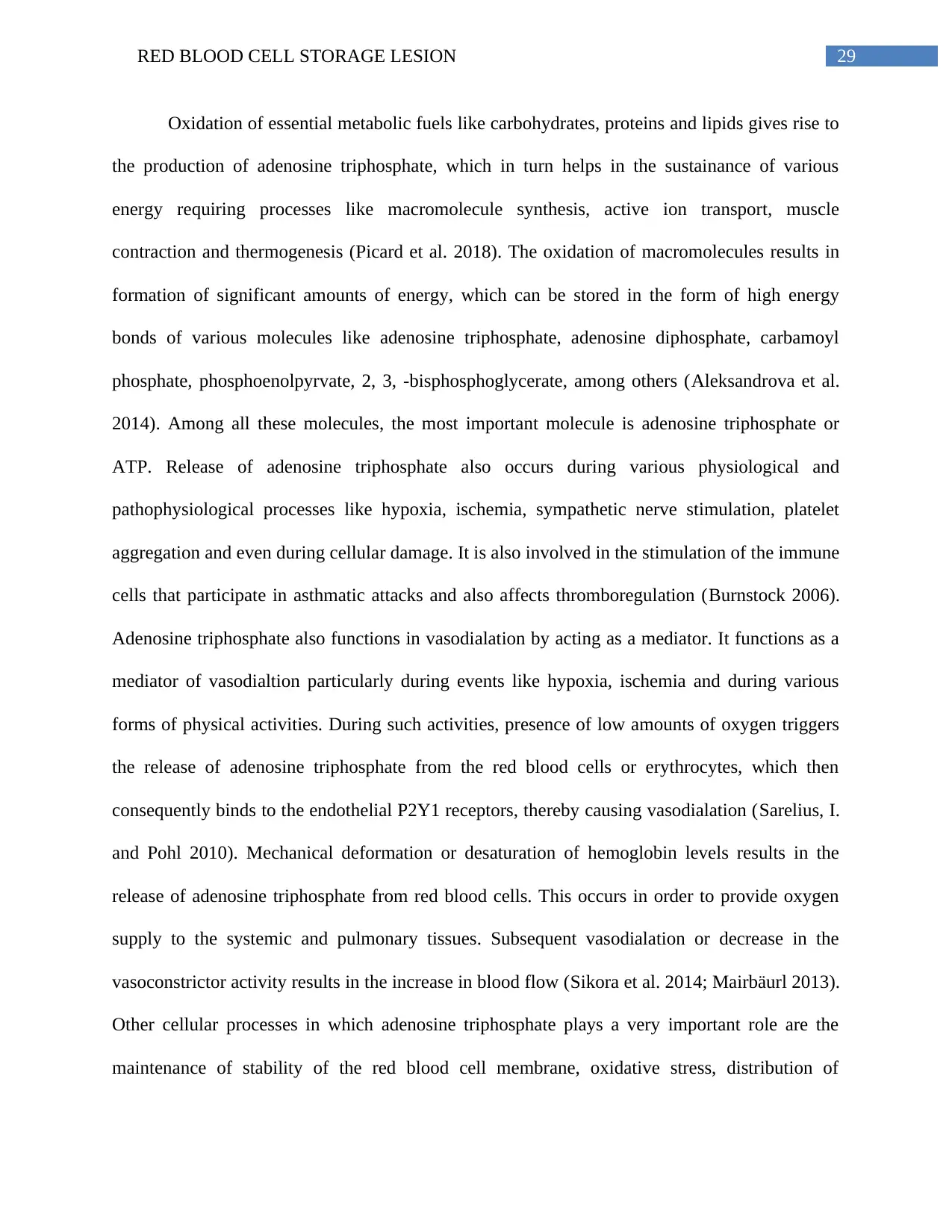
29RED BLOOD CELL STORAGE LESION
Oxidation of essential metabolic fuels like carbohydrates, proteins and lipids gives rise to
the production of adenosine triphosphate, which in turn helps in the sustainance of various
energy requiring processes like macromolecule synthesis, active ion transport, muscle
contraction and thermogenesis (Picard et al. 2018). The oxidation of macromolecules results in
formation of significant amounts of energy, which can be stored in the form of high energy
bonds of various molecules like adenosine triphosphate, adenosine diphosphate, carbamoyl
phosphate, phosphoenolpyrvate, 2, 3, -bisphosphoglycerate, among others (Aleksandrova et al.
2014). Among all these molecules, the most important molecule is adenosine triphosphate or
ATP. Release of adenosine triphosphate also occurs during various physiological and
pathophysiological processes like hypoxia, ischemia, sympathetic nerve stimulation, platelet
aggregation and even during cellular damage. It is also involved in the stimulation of the immune
cells that participate in asthmatic attacks and also affects thromboregulation (Burnstock 2006).
Adenosine triphosphate also functions in vasodialation by acting as a mediator. It functions as a
mediator of vasodialtion particularly during events like hypoxia, ischemia and during various
forms of physical activities. During such activities, presence of low amounts of oxygen triggers
the release of adenosine triphosphate from the red blood cells or erythrocytes, which then
consequently binds to the endothelial P2Y1 receptors, thereby causing vasodialation (Sarelius, I.
and Pohl 2010). Mechanical deformation or desaturation of hemoglobin levels results in the
release of adenosine triphosphate from red blood cells. This occurs in order to provide oxygen
supply to the systemic and pulmonary tissues. Subsequent vasodialation or decrease in the
vasoconstrictor activity results in the increase in blood flow (Sikora et al. 2014; Mairbäurl 2013).
Other cellular processes in which adenosine triphosphate plays a very important role are the
maintenance of stability of the red blood cell membrane, oxidative stress, distribution of
Oxidation of essential metabolic fuels like carbohydrates, proteins and lipids gives rise to
the production of adenosine triphosphate, which in turn helps in the sustainance of various
energy requiring processes like macromolecule synthesis, active ion transport, muscle
contraction and thermogenesis (Picard et al. 2018). The oxidation of macromolecules results in
formation of significant amounts of energy, which can be stored in the form of high energy
bonds of various molecules like adenosine triphosphate, adenosine diphosphate, carbamoyl
phosphate, phosphoenolpyrvate, 2, 3, -bisphosphoglycerate, among others (Aleksandrova et al.
2014). Among all these molecules, the most important molecule is adenosine triphosphate or
ATP. Release of adenosine triphosphate also occurs during various physiological and
pathophysiological processes like hypoxia, ischemia, sympathetic nerve stimulation, platelet
aggregation and even during cellular damage. It is also involved in the stimulation of the immune
cells that participate in asthmatic attacks and also affects thromboregulation (Burnstock 2006).
Adenosine triphosphate also functions in vasodialation by acting as a mediator. It functions as a
mediator of vasodialtion particularly during events like hypoxia, ischemia and during various
forms of physical activities. During such activities, presence of low amounts of oxygen triggers
the release of adenosine triphosphate from the red blood cells or erythrocytes, which then
consequently binds to the endothelial P2Y1 receptors, thereby causing vasodialation (Sarelius, I.
and Pohl 2010). Mechanical deformation or desaturation of hemoglobin levels results in the
release of adenosine triphosphate from red blood cells. This occurs in order to provide oxygen
supply to the systemic and pulmonary tissues. Subsequent vasodialation or decrease in the
vasoconstrictor activity results in the increase in blood flow (Sikora et al. 2014; Mairbäurl 2013).
Other cellular processes in which adenosine triphosphate plays a very important role are the
maintenance of stability of the red blood cell membrane, oxidative stress, distribution of
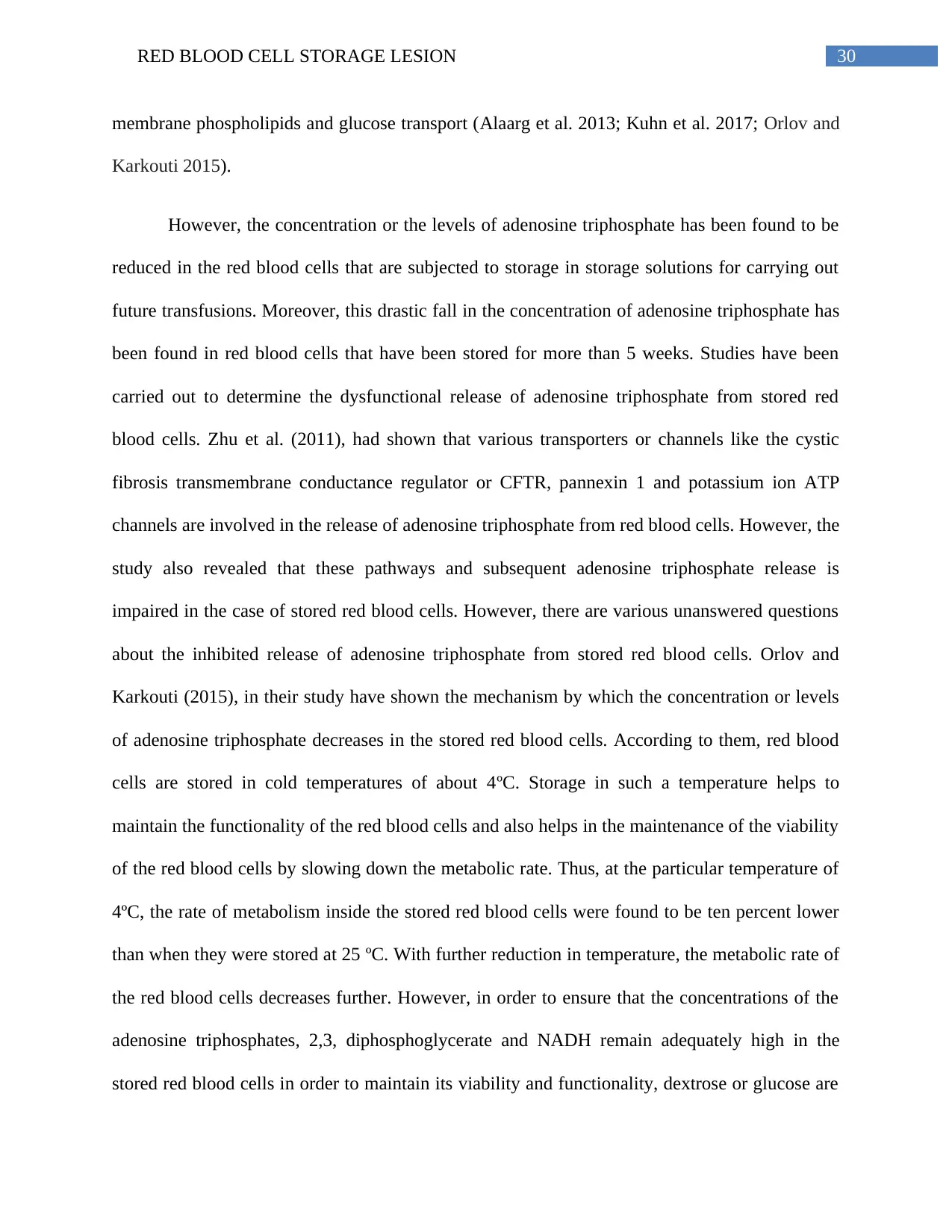
30RED BLOOD CELL STORAGE LESION
membrane phospholipids and glucose transport (Alaarg et al. 2013; Kuhn et al. 2017; Orlov and
Karkouti 2015).
However, the concentration or the levels of adenosine triphosphate has been found to be
reduced in the red blood cells that are subjected to storage in storage solutions for carrying out
future transfusions. Moreover, this drastic fall in the concentration of adenosine triphosphate has
been found in red blood cells that have been stored for more than 5 weeks. Studies have been
carried out to determine the dysfunctional release of adenosine triphosphate from stored red
blood cells. Zhu et al. (2011), had shown that various transporters or channels like the cystic
fibrosis transmembrane conductance regulator or CFTR, pannexin 1 and potassium ion ATP
channels are involved in the release of adenosine triphosphate from red blood cells. However, the
study also revealed that these pathways and subsequent adenosine triphosphate release is
impaired in the case of stored red blood cells. However, there are various unanswered questions
about the inhibited release of adenosine triphosphate from stored red blood cells. Orlov and
Karkouti (2015), in their study have shown the mechanism by which the concentration or levels
of adenosine triphosphate decreases in the stored red blood cells. According to them, red blood
cells are stored in cold temperatures of about 4ºC. Storage in such a temperature helps to
maintain the functionality of the red blood cells and also helps in the maintenance of the viability
of the red blood cells by slowing down the metabolic rate. Thus, at the particular temperature of
4ºC, the rate of metabolism inside the stored red blood cells were found to be ten percent lower
than when they were stored at 25 ºC. With further reduction in temperature, the metabolic rate of
the red blood cells decreases further. However, in order to ensure that the concentrations of the
adenosine triphosphates, 2,3, diphosphoglycerate and NADH remain adequately high in the
stored red blood cells in order to maintain its viability and functionality, dextrose or glucose are
membrane phospholipids and glucose transport (Alaarg et al. 2013; Kuhn et al. 2017; Orlov and
Karkouti 2015).
However, the concentration or the levels of adenosine triphosphate has been found to be
reduced in the red blood cells that are subjected to storage in storage solutions for carrying out
future transfusions. Moreover, this drastic fall in the concentration of adenosine triphosphate has
been found in red blood cells that have been stored for more than 5 weeks. Studies have been
carried out to determine the dysfunctional release of adenosine triphosphate from stored red
blood cells. Zhu et al. (2011), had shown that various transporters or channels like the cystic
fibrosis transmembrane conductance regulator or CFTR, pannexin 1 and potassium ion ATP
channels are involved in the release of adenosine triphosphate from red blood cells. However, the
study also revealed that these pathways and subsequent adenosine triphosphate release is
impaired in the case of stored red blood cells. However, there are various unanswered questions
about the inhibited release of adenosine triphosphate from stored red blood cells. Orlov and
Karkouti (2015), in their study have shown the mechanism by which the concentration or levels
of adenosine triphosphate decreases in the stored red blood cells. According to them, red blood
cells are stored in cold temperatures of about 4ºC. Storage in such a temperature helps to
maintain the functionality of the red blood cells and also helps in the maintenance of the viability
of the red blood cells by slowing down the metabolic rate. Thus, at the particular temperature of
4ºC, the rate of metabolism inside the stored red blood cells were found to be ten percent lower
than when they were stored at 25 ºC. With further reduction in temperature, the metabolic rate of
the red blood cells decreases further. However, in order to ensure that the concentrations of the
adenosine triphosphates, 2,3, diphosphoglycerate and NADH remain adequately high in the
stored red blood cells in order to maintain its viability and functionality, dextrose or glucose are
Paraphrase This Document
Need a fresh take? Get an instant paraphrase of this document with our AI Paraphraser
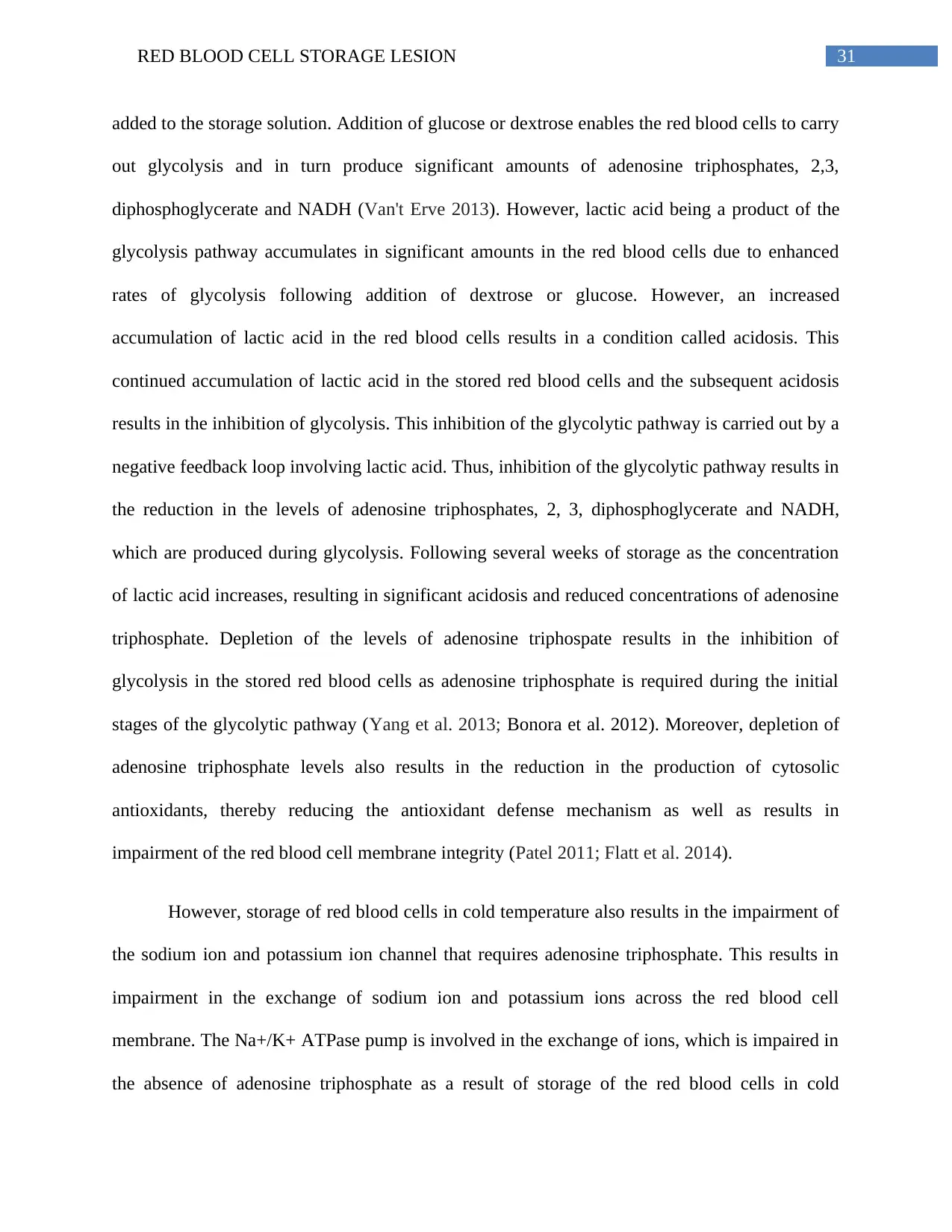
31RED BLOOD CELL STORAGE LESION
added to the storage solution. Addition of glucose or dextrose enables the red blood cells to carry
out glycolysis and in turn produce significant amounts of adenosine triphosphates, 2,3,
diphosphoglycerate and NADH (Van't Erve 2013). However, lactic acid being a product of the
glycolysis pathway accumulates in significant amounts in the red blood cells due to enhanced
rates of glycolysis following addition of dextrose or glucose. However, an increased
accumulation of lactic acid in the red blood cells results in a condition called acidosis. This
continued accumulation of lactic acid in the stored red blood cells and the subsequent acidosis
results in the inhibition of glycolysis. This inhibition of the glycolytic pathway is carried out by a
negative feedback loop involving lactic acid. Thus, inhibition of the glycolytic pathway results in
the reduction in the levels of adenosine triphosphates, 2, 3, diphosphoglycerate and NADH,
which are produced during glycolysis. Following several weeks of storage as the concentration
of lactic acid increases, resulting in significant acidosis and reduced concentrations of adenosine
triphosphate. Depletion of the levels of adenosine triphospate results in the inhibition of
glycolysis in the stored red blood cells as adenosine triphosphate is required during the initial
stages of the glycolytic pathway (Yang et al. 2013; Bonora et al. 2012). Moreover, depletion of
adenosine triphosphate levels also results in the reduction in the production of cytosolic
antioxidants, thereby reducing the antioxidant defense mechanism as well as results in
impairment of the red blood cell membrane integrity (Patel 2011; Flatt et al. 2014).
However, storage of red blood cells in cold temperature also results in the impairment of
the sodium ion and potassium ion channel that requires adenosine triphosphate. This results in
impairment in the exchange of sodium ion and potassium ions across the red blood cell
membrane. The Na+/K+ ATPase pump is involved in the exchange of ions, which is impaired in
the absence of adenosine triphosphate as a result of storage of the red blood cells in cold
added to the storage solution. Addition of glucose or dextrose enables the red blood cells to carry
out glycolysis and in turn produce significant amounts of adenosine triphosphates, 2,3,
diphosphoglycerate and NADH (Van't Erve 2013). However, lactic acid being a product of the
glycolysis pathway accumulates in significant amounts in the red blood cells due to enhanced
rates of glycolysis following addition of dextrose or glucose. However, an increased
accumulation of lactic acid in the red blood cells results in a condition called acidosis. This
continued accumulation of lactic acid in the stored red blood cells and the subsequent acidosis
results in the inhibition of glycolysis. This inhibition of the glycolytic pathway is carried out by a
negative feedback loop involving lactic acid. Thus, inhibition of the glycolytic pathway results in
the reduction in the levels of adenosine triphosphates, 2, 3, diphosphoglycerate and NADH,
which are produced during glycolysis. Following several weeks of storage as the concentration
of lactic acid increases, resulting in significant acidosis and reduced concentrations of adenosine
triphosphate. Depletion of the levels of adenosine triphospate results in the inhibition of
glycolysis in the stored red blood cells as adenosine triphosphate is required during the initial
stages of the glycolytic pathway (Yang et al. 2013; Bonora et al. 2012). Moreover, depletion of
adenosine triphosphate levels also results in the reduction in the production of cytosolic
antioxidants, thereby reducing the antioxidant defense mechanism as well as results in
impairment of the red blood cell membrane integrity (Patel 2011; Flatt et al. 2014).
However, storage of red blood cells in cold temperature also results in the impairment of
the sodium ion and potassium ion channel that requires adenosine triphosphate. This results in
impairment in the exchange of sodium ion and potassium ions across the red blood cell
membrane. The Na+/K+ ATPase pump is involved in the exchange of ions, which is impaired in
the absence of adenosine triphosphate as a result of storage of the red blood cells in cold
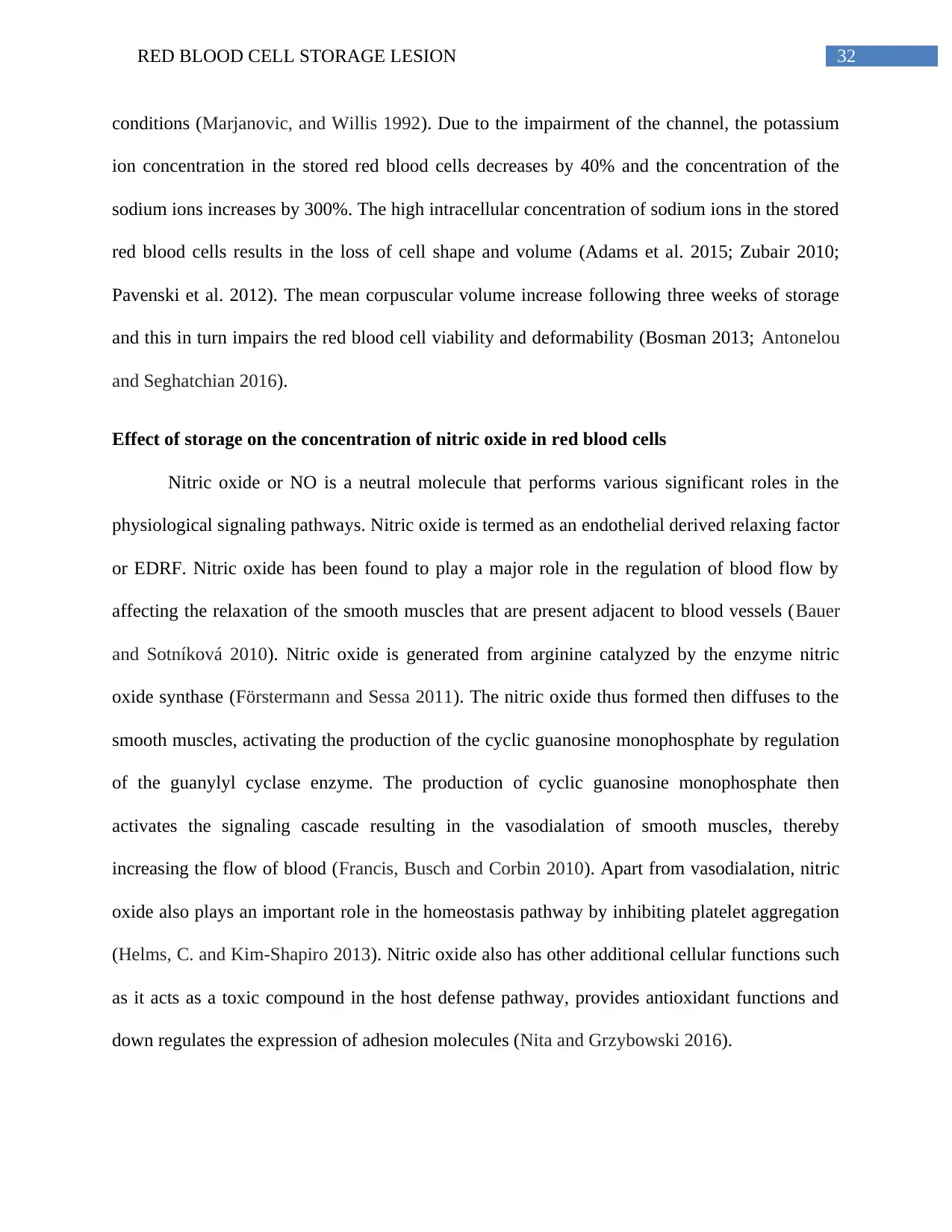
32RED BLOOD CELL STORAGE LESION
conditions (Marjanovic, and Willis 1992). Due to the impairment of the channel, the potassium
ion concentration in the stored red blood cells decreases by 40% and the concentration of the
sodium ions increases by 300%. The high intracellular concentration of sodium ions in the stored
red blood cells results in the loss of cell shape and volume (Adams et al. 2015; Zubair 2010;
Pavenski et al. 2012). The mean corpuscular volume increase following three weeks of storage
and this in turn impairs the red blood cell viability and deformability (Bosman 2013; Antonelou
and Seghatchian 2016).
Effect of storage on the concentration of nitric oxide in red blood cells
Nitric oxide or NO is a neutral molecule that performs various significant roles in the
physiological signaling pathways. Nitric oxide is termed as an endothelial derived relaxing factor
or EDRF. Nitric oxide has been found to play a major role in the regulation of blood flow by
affecting the relaxation of the smooth muscles that are present adjacent to blood vessels (Bauer
and Sotníková 2010). Nitric oxide is generated from arginine catalyzed by the enzyme nitric
oxide synthase (Förstermann and Sessa 2011). The nitric oxide thus formed then diffuses to the
smooth muscles, activating the production of the cyclic guanosine monophosphate by regulation
of the guanylyl cyclase enzyme. The production of cyclic guanosine monophosphate then
activates the signaling cascade resulting in the vasodialation of smooth muscles, thereby
increasing the flow of blood (Francis, Busch and Corbin 2010). Apart from vasodialation, nitric
oxide also plays an important role in the homeostasis pathway by inhibiting platelet aggregation
(Helms, C. and Kim-Shapiro 2013). Nitric oxide also has other additional cellular functions such
as it acts as a toxic compound in the host defense pathway, provides antioxidant functions and
down regulates the expression of adhesion molecules (Nita and Grzybowski 2016).
conditions (Marjanovic, and Willis 1992). Due to the impairment of the channel, the potassium
ion concentration in the stored red blood cells decreases by 40% and the concentration of the
sodium ions increases by 300%. The high intracellular concentration of sodium ions in the stored
red blood cells results in the loss of cell shape and volume (Adams et al. 2015; Zubair 2010;
Pavenski et al. 2012). The mean corpuscular volume increase following three weeks of storage
and this in turn impairs the red blood cell viability and deformability (Bosman 2013; Antonelou
and Seghatchian 2016).
Effect of storage on the concentration of nitric oxide in red blood cells
Nitric oxide or NO is a neutral molecule that performs various significant roles in the
physiological signaling pathways. Nitric oxide is termed as an endothelial derived relaxing factor
or EDRF. Nitric oxide has been found to play a major role in the regulation of blood flow by
affecting the relaxation of the smooth muscles that are present adjacent to blood vessels (Bauer
and Sotníková 2010). Nitric oxide is generated from arginine catalyzed by the enzyme nitric
oxide synthase (Förstermann and Sessa 2011). The nitric oxide thus formed then diffuses to the
smooth muscles, activating the production of the cyclic guanosine monophosphate by regulation
of the guanylyl cyclase enzyme. The production of cyclic guanosine monophosphate then
activates the signaling cascade resulting in the vasodialation of smooth muscles, thereby
increasing the flow of blood (Francis, Busch and Corbin 2010). Apart from vasodialation, nitric
oxide also plays an important role in the homeostasis pathway by inhibiting platelet aggregation
(Helms, C. and Kim-Shapiro 2013). Nitric oxide also has other additional cellular functions such
as it acts as a toxic compound in the host defense pathway, provides antioxidant functions and
down regulates the expression of adhesion molecules (Nita and Grzybowski 2016).
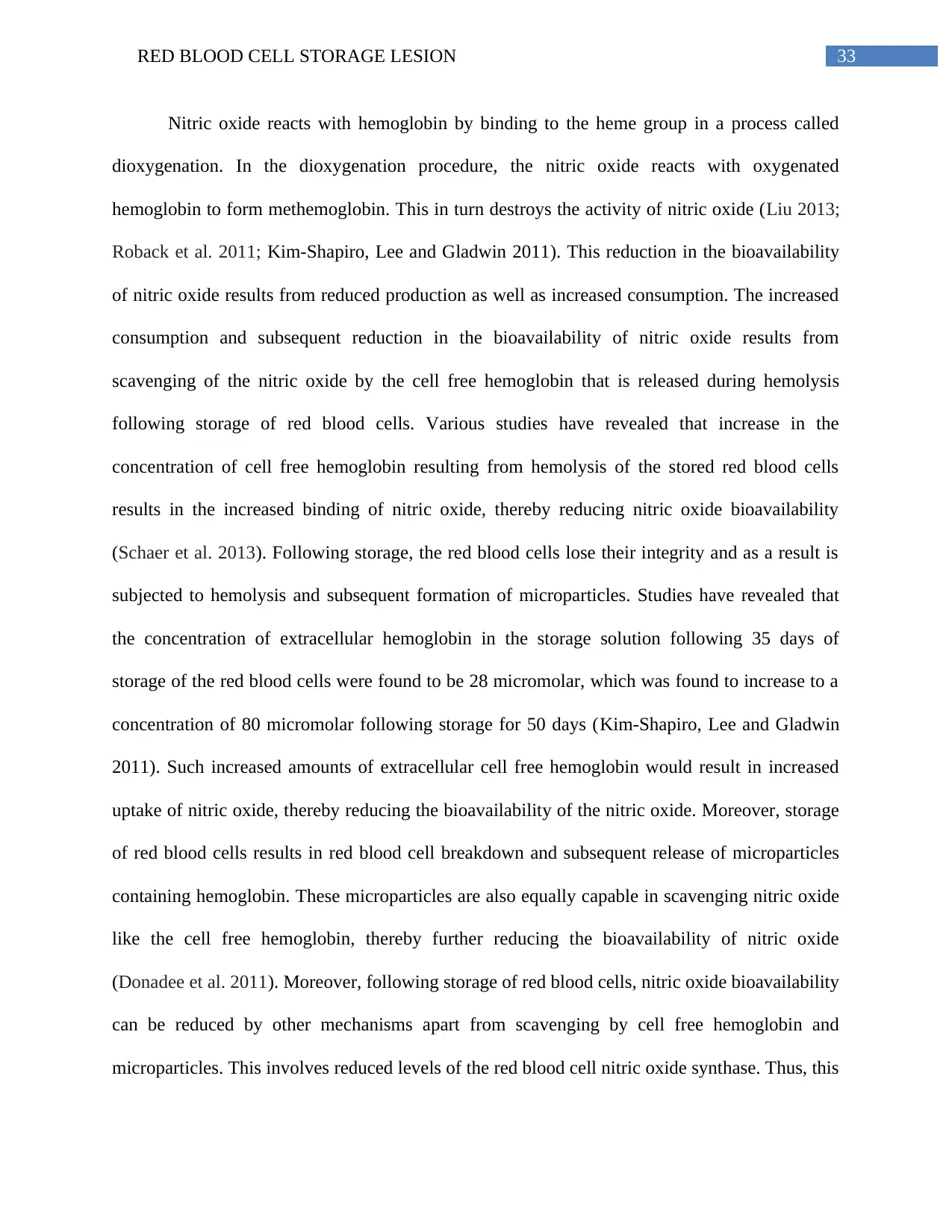
33RED BLOOD CELL STORAGE LESION
Nitric oxide reacts with hemoglobin by binding to the heme group in a process called
dioxygenation. In the dioxygenation procedure, the nitric oxide reacts with oxygenated
hemoglobin to form methemoglobin. This in turn destroys the activity of nitric oxide (Liu 2013;
Roback et al. 2011; Kim‐Shapiro, Lee and Gladwin 2011). This reduction in the bioavailability
of nitric oxide results from reduced production as well as increased consumption. The increased
consumption and subsequent reduction in the bioavailability of nitric oxide results from
scavenging of the nitric oxide by the cell free hemoglobin that is released during hemolysis
following storage of red blood cells. Various studies have revealed that increase in the
concentration of cell free hemoglobin resulting from hemolysis of the stored red blood cells
results in the increased binding of nitric oxide, thereby reducing nitric oxide bioavailability
(Schaer et al. 2013). Following storage, the red blood cells lose their integrity and as a result is
subjected to hemolysis and subsequent formation of microparticles. Studies have revealed that
the concentration of extracellular hemoglobin in the storage solution following 35 days of
storage of the red blood cells were found to be 28 micromolar, which was found to increase to a
concentration of 80 micromolar following storage for 50 days (Kim‐Shapiro, Lee and Gladwin
2011). Such increased amounts of extracellular cell free hemoglobin would result in increased
uptake of nitric oxide, thereby reducing the bioavailability of the nitric oxide. Moreover, storage
of red blood cells results in red blood cell breakdown and subsequent release of microparticles
containing hemoglobin. These microparticles are also equally capable in scavenging nitric oxide
like the cell free hemoglobin, thereby further reducing the bioavailability of nitric oxide
(Donadee et al. 2011). Moreover, following storage of red blood cells, nitric oxide bioavailability
can be reduced by other mechanisms apart from scavenging by cell free hemoglobin and
microparticles. This involves reduced levels of the red blood cell nitric oxide synthase. Thus, this
Nitric oxide reacts with hemoglobin by binding to the heme group in a process called
dioxygenation. In the dioxygenation procedure, the nitric oxide reacts with oxygenated
hemoglobin to form methemoglobin. This in turn destroys the activity of nitric oxide (Liu 2013;
Roback et al. 2011; Kim‐Shapiro, Lee and Gladwin 2011). This reduction in the bioavailability
of nitric oxide results from reduced production as well as increased consumption. The increased
consumption and subsequent reduction in the bioavailability of nitric oxide results from
scavenging of the nitric oxide by the cell free hemoglobin that is released during hemolysis
following storage of red blood cells. Various studies have revealed that increase in the
concentration of cell free hemoglobin resulting from hemolysis of the stored red blood cells
results in the increased binding of nitric oxide, thereby reducing nitric oxide bioavailability
(Schaer et al. 2013). Following storage, the red blood cells lose their integrity and as a result is
subjected to hemolysis and subsequent formation of microparticles. Studies have revealed that
the concentration of extracellular hemoglobin in the storage solution following 35 days of
storage of the red blood cells were found to be 28 micromolar, which was found to increase to a
concentration of 80 micromolar following storage for 50 days (Kim‐Shapiro, Lee and Gladwin
2011). Such increased amounts of extracellular cell free hemoglobin would result in increased
uptake of nitric oxide, thereby reducing the bioavailability of the nitric oxide. Moreover, storage
of red blood cells results in red blood cell breakdown and subsequent release of microparticles
containing hemoglobin. These microparticles are also equally capable in scavenging nitric oxide
like the cell free hemoglobin, thereby further reducing the bioavailability of nitric oxide
(Donadee et al. 2011). Moreover, following storage of red blood cells, nitric oxide bioavailability
can be reduced by other mechanisms apart from scavenging by cell free hemoglobin and
microparticles. This involves reduced levels of the red blood cell nitric oxide synthase. Thus, this
Secure Best Marks with AI Grader
Need help grading? Try our AI Grader for instant feedback on your assignments.
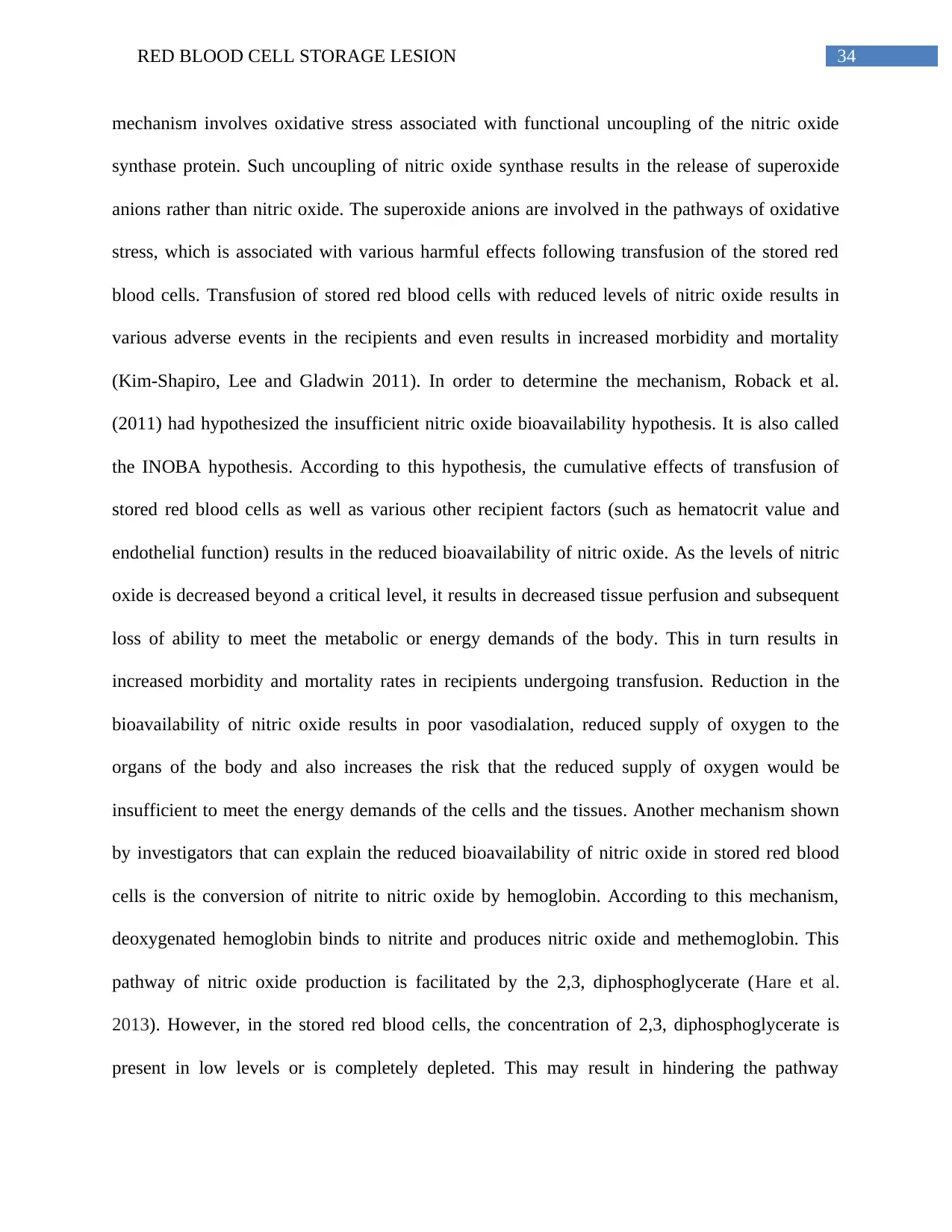
34RED BLOOD CELL STORAGE LESION
mechanism involves oxidative stress associated with functional uncoupling of the nitric oxide
synthase protein. Such uncoupling of nitric oxide synthase results in the release of superoxide
anions rather than nitric oxide. The superoxide anions are involved in the pathways of oxidative
stress, which is associated with various harmful effects following transfusion of the stored red
blood cells. Transfusion of stored red blood cells with reduced levels of nitric oxide results in
various adverse events in the recipients and even results in increased morbidity and mortality
(Kim‐Shapiro, Lee and Gladwin 2011). In order to determine the mechanism, Roback et al.
(2011) had hypothesized the insufficient nitric oxide bioavailability hypothesis. It is also called
the INOBA hypothesis. According to this hypothesis, the cumulative effects of transfusion of
stored red blood cells as well as various other recipient factors (such as hematocrit value and
endothelial function) results in the reduced bioavailability of nitric oxide. As the levels of nitric
oxide is decreased beyond a critical level, it results in decreased tissue perfusion and subsequent
loss of ability to meet the metabolic or energy demands of the body. This in turn results in
increased morbidity and mortality rates in recipients undergoing transfusion. Reduction in the
bioavailability of nitric oxide results in poor vasodialation, reduced supply of oxygen to the
organs of the body and also increases the risk that the reduced supply of oxygen would be
insufficient to meet the energy demands of the cells and the tissues. Another mechanism shown
by investigators that can explain the reduced bioavailability of nitric oxide in stored red blood
cells is the conversion of nitrite to nitric oxide by hemoglobin. According to this mechanism,
deoxygenated hemoglobin binds to nitrite and produces nitric oxide and methemoglobin. This
pathway of nitric oxide production is facilitated by the 2,3, diphosphoglycerate (Hare et al.
2013). However, in the stored red blood cells, the concentration of 2,3, diphosphoglycerate is
present in low levels or is completely depleted. This may result in hindering the pathway
mechanism involves oxidative stress associated with functional uncoupling of the nitric oxide
synthase protein. Such uncoupling of nitric oxide synthase results in the release of superoxide
anions rather than nitric oxide. The superoxide anions are involved in the pathways of oxidative
stress, which is associated with various harmful effects following transfusion of the stored red
blood cells. Transfusion of stored red blood cells with reduced levels of nitric oxide results in
various adverse events in the recipients and even results in increased morbidity and mortality
(Kim‐Shapiro, Lee and Gladwin 2011). In order to determine the mechanism, Roback et al.
(2011) had hypothesized the insufficient nitric oxide bioavailability hypothesis. It is also called
the INOBA hypothesis. According to this hypothesis, the cumulative effects of transfusion of
stored red blood cells as well as various other recipient factors (such as hematocrit value and
endothelial function) results in the reduced bioavailability of nitric oxide. As the levels of nitric
oxide is decreased beyond a critical level, it results in decreased tissue perfusion and subsequent
loss of ability to meet the metabolic or energy demands of the body. This in turn results in
increased morbidity and mortality rates in recipients undergoing transfusion. Reduction in the
bioavailability of nitric oxide results in poor vasodialation, reduced supply of oxygen to the
organs of the body and also increases the risk that the reduced supply of oxygen would be
insufficient to meet the energy demands of the cells and the tissues. Another mechanism shown
by investigators that can explain the reduced bioavailability of nitric oxide in stored red blood
cells is the conversion of nitrite to nitric oxide by hemoglobin. According to this mechanism,
deoxygenated hemoglobin binds to nitrite and produces nitric oxide and methemoglobin. This
pathway of nitric oxide production is facilitated by the 2,3, diphosphoglycerate (Hare et al.
2013). However, in the stored red blood cells, the concentration of 2,3, diphosphoglycerate is
present in low levels or is completely depleted. This may result in hindering the pathway
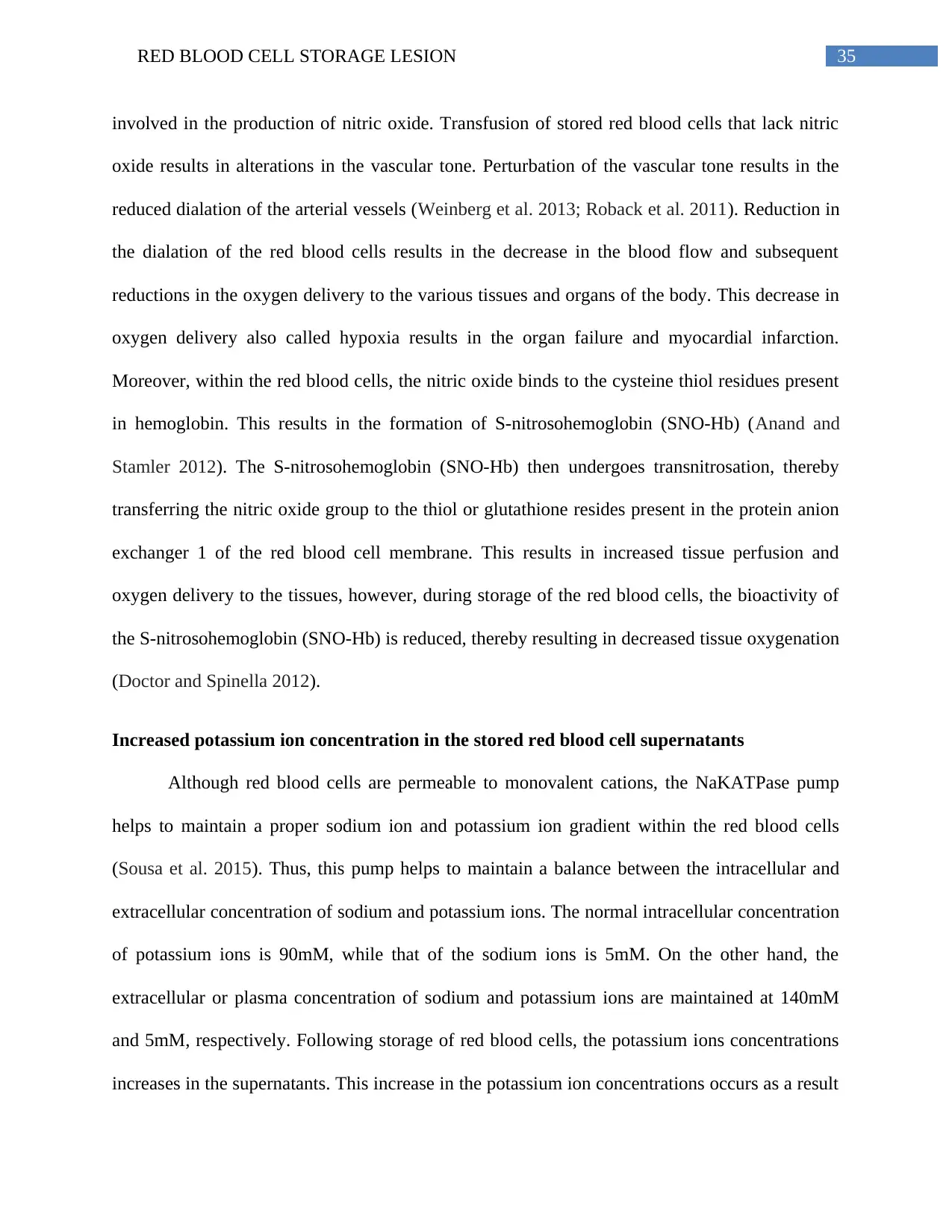
35RED BLOOD CELL STORAGE LESION
involved in the production of nitric oxide. Transfusion of stored red blood cells that lack nitric
oxide results in alterations in the vascular tone. Perturbation of the vascular tone results in the
reduced dialation of the arterial vessels (Weinberg et al. 2013; Roback et al. 2011). Reduction in
the dialation of the red blood cells results in the decrease in the blood flow and subsequent
reductions in the oxygen delivery to the various tissues and organs of the body. This decrease in
oxygen delivery also called hypoxia results in the organ failure and myocardial infarction.
Moreover, within the red blood cells, the nitric oxide binds to the cysteine thiol residues present
in hemoglobin. This results in the formation of S-nitrosohemoglobin (SNO-Hb) (Anand and
Stamler 2012). The S-nitrosohemoglobin (SNO-Hb) then undergoes transnitrosation, thereby
transferring the nitric oxide group to the thiol or glutathione resides present in the protein anion
exchanger 1 of the red blood cell membrane. This results in increased tissue perfusion and
oxygen delivery to the tissues, however, during storage of the red blood cells, the bioactivity of
the S-nitrosohemoglobin (SNO-Hb) is reduced, thereby resulting in decreased tissue oxygenation
(Doctor and Spinella 2012).
Increased potassium ion concentration in the stored red blood cell supernatants
Although red blood cells are permeable to monovalent cations, the NaKATPase pump
helps to maintain a proper sodium ion and potassium ion gradient within the red blood cells
(Sousa et al. 2015). Thus, this pump helps to maintain a balance between the intracellular and
extracellular concentration of sodium and potassium ions. The normal intracellular concentration
of potassium ions is 90mM, while that of the sodium ions is 5mM. On the other hand, the
extracellular or plasma concentration of sodium and potassium ions are maintained at 140mM
and 5mM, respectively. Following storage of red blood cells, the potassium ions concentrations
increases in the supernatants. This increase in the potassium ion concentrations occurs as a result
involved in the production of nitric oxide. Transfusion of stored red blood cells that lack nitric
oxide results in alterations in the vascular tone. Perturbation of the vascular tone results in the
reduced dialation of the arterial vessels (Weinberg et al. 2013; Roback et al. 2011). Reduction in
the dialation of the red blood cells results in the decrease in the blood flow and subsequent
reductions in the oxygen delivery to the various tissues and organs of the body. This decrease in
oxygen delivery also called hypoxia results in the organ failure and myocardial infarction.
Moreover, within the red blood cells, the nitric oxide binds to the cysteine thiol residues present
in hemoglobin. This results in the formation of S-nitrosohemoglobin (SNO-Hb) (Anand and
Stamler 2012). The S-nitrosohemoglobin (SNO-Hb) then undergoes transnitrosation, thereby
transferring the nitric oxide group to the thiol or glutathione resides present in the protein anion
exchanger 1 of the red blood cell membrane. This results in increased tissue perfusion and
oxygen delivery to the tissues, however, during storage of the red blood cells, the bioactivity of
the S-nitrosohemoglobin (SNO-Hb) is reduced, thereby resulting in decreased tissue oxygenation
(Doctor and Spinella 2012).
Increased potassium ion concentration in the stored red blood cell supernatants
Although red blood cells are permeable to monovalent cations, the NaKATPase pump
helps to maintain a proper sodium ion and potassium ion gradient within the red blood cells
(Sousa et al. 2015). Thus, this pump helps to maintain a balance between the intracellular and
extracellular concentration of sodium and potassium ions. The normal intracellular concentration
of potassium ions is 90mM, while that of the sodium ions is 5mM. On the other hand, the
extracellular or plasma concentration of sodium and potassium ions are maintained at 140mM
and 5mM, respectively. Following storage of red blood cells, the potassium ions concentrations
increases in the supernatants. This increase in the potassium ion concentrations occurs as a result
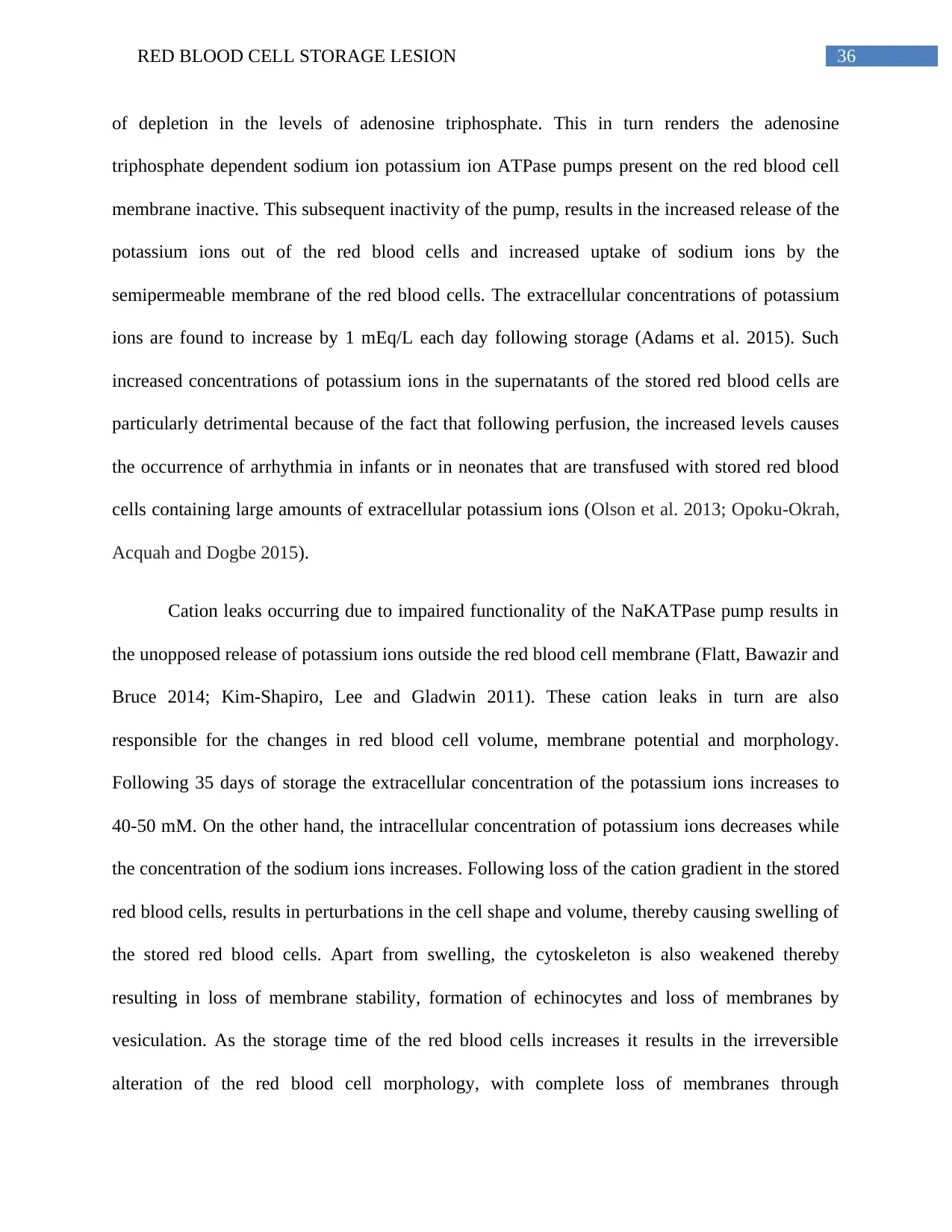
36RED BLOOD CELL STORAGE LESION
of depletion in the levels of adenosine triphosphate. This in turn renders the adenosine
triphosphate dependent sodium ion potassium ion ATPase pumps present on the red blood cell
membrane inactive. This subsequent inactivity of the pump, results in the increased release of the
potassium ions out of the red blood cells and increased uptake of sodium ions by the
semipermeable membrane of the red blood cells. The extracellular concentrations of potassium
ions are found to increase by 1 mEq/L each day following storage (Adams et al. 2015). Such
increased concentrations of potassium ions in the supernatants of the stored red blood cells are
particularly detrimental because of the fact that following perfusion, the increased levels causes
the occurrence of arrhythmia in infants or in neonates that are transfused with stored red blood
cells containing large amounts of extracellular potassium ions (Olson et al. 2013; Opoku-Okrah,
Acquah and Dogbe 2015).
Cation leaks occurring due to impaired functionality of the NaKATPase pump results in
the unopposed release of potassium ions outside the red blood cell membrane (Flatt, Bawazir and
Bruce 2014; Kim‐Shapiro, Lee and Gladwin 2011). These cation leaks in turn are also
responsible for the changes in red blood cell volume, membrane potential and morphology.
Following 35 days of storage the extracellular concentration of the potassium ions increases to
40-50 mM. On the other hand, the intracellular concentration of potassium ions decreases while
the concentration of the sodium ions increases. Following loss of the cation gradient in the stored
red blood cells, results in perturbations in the cell shape and volume, thereby causing swelling of
the stored red blood cells. Apart from swelling, the cytoskeleton is also weakened thereby
resulting in loss of membrane stability, formation of echinocytes and loss of membranes by
vesiculation. As the storage time of the red blood cells increases it results in the irreversible
alteration of the red blood cell morphology, with complete loss of membranes through
of depletion in the levels of adenosine triphosphate. This in turn renders the adenosine
triphosphate dependent sodium ion potassium ion ATPase pumps present on the red blood cell
membrane inactive. This subsequent inactivity of the pump, results in the increased release of the
potassium ions out of the red blood cells and increased uptake of sodium ions by the
semipermeable membrane of the red blood cells. The extracellular concentrations of potassium
ions are found to increase by 1 mEq/L each day following storage (Adams et al. 2015). Such
increased concentrations of potassium ions in the supernatants of the stored red blood cells are
particularly detrimental because of the fact that following perfusion, the increased levels causes
the occurrence of arrhythmia in infants or in neonates that are transfused with stored red blood
cells containing large amounts of extracellular potassium ions (Olson et al. 2013; Opoku-Okrah,
Acquah and Dogbe 2015).
Cation leaks occurring due to impaired functionality of the NaKATPase pump results in
the unopposed release of potassium ions outside the red blood cell membrane (Flatt, Bawazir and
Bruce 2014; Kim‐Shapiro, Lee and Gladwin 2011). These cation leaks in turn are also
responsible for the changes in red blood cell volume, membrane potential and morphology.
Following 35 days of storage the extracellular concentration of the potassium ions increases to
40-50 mM. On the other hand, the intracellular concentration of potassium ions decreases while
the concentration of the sodium ions increases. Following loss of the cation gradient in the stored
red blood cells, results in perturbations in the cell shape and volume, thereby causing swelling of
the stored red blood cells. Apart from swelling, the cytoskeleton is also weakened thereby
resulting in loss of membrane stability, formation of echinocytes and loss of membranes by
vesiculation. As the storage time of the red blood cells increases it results in the irreversible
alteration of the red blood cell morphology, with complete loss of membranes through
Paraphrase This Document
Need a fresh take? Get an instant paraphrase of this document with our AI Paraphraser
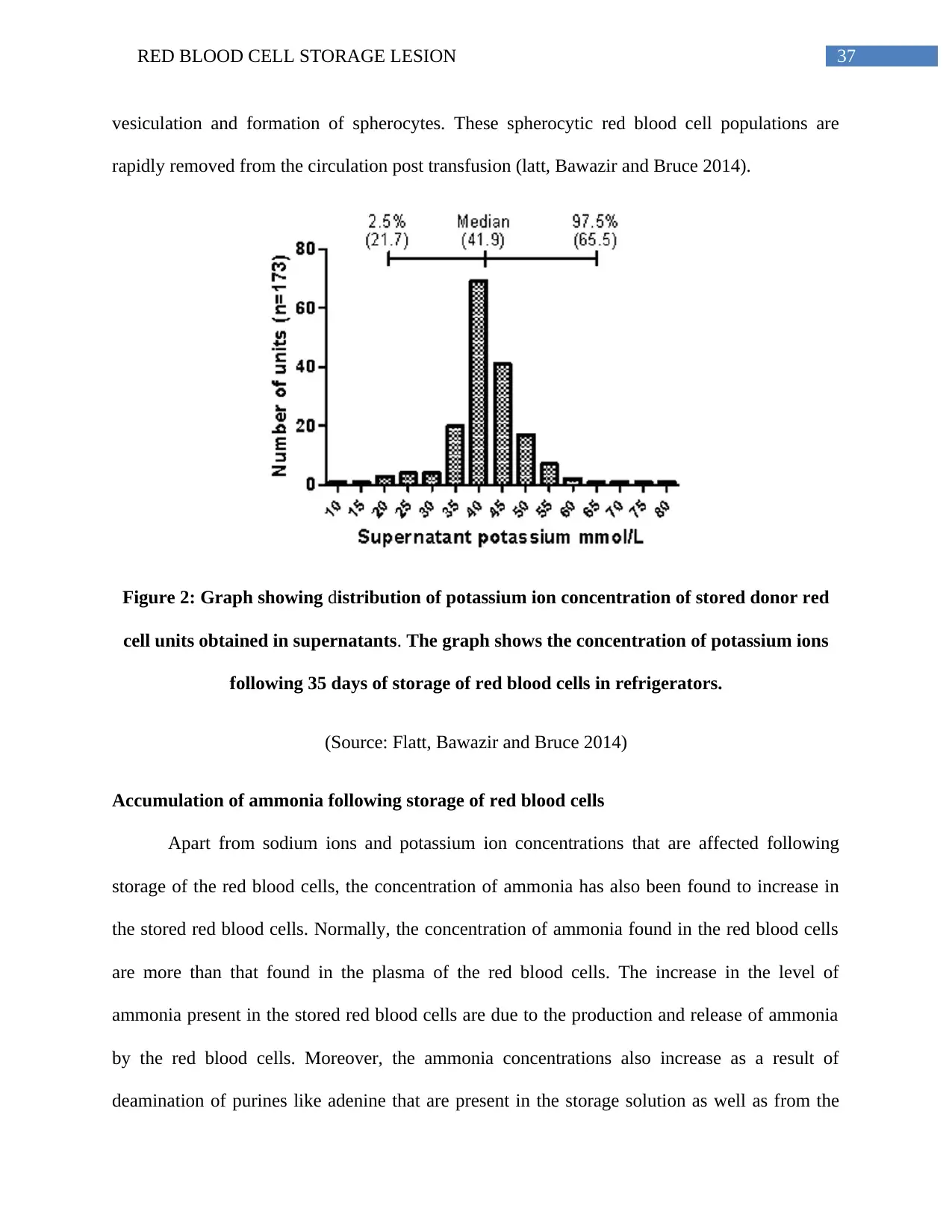
37RED BLOOD CELL STORAGE LESION
vesiculation and formation of spherocytes. These spherocytic red blood cell populations are
rapidly removed from the circulation post transfusion (latt, Bawazir and Bruce 2014).
Figure 2: Graph showing distribution of potassium ion concentration of stored donor red
cell units obtained in supernatants. The graph shows the concentration of potassium ions
following 35 days of storage of red blood cells in refrigerators.
(Source: Flatt, Bawazir and Bruce 2014)
Accumulation of ammonia following storage of red blood cells
Apart from sodium ions and potassium ion concentrations that are affected following
storage of the red blood cells, the concentration of ammonia has also been found to increase in
the stored red blood cells. Normally, the concentration of ammonia found in the red blood cells
are more than that found in the plasma of the red blood cells. The increase in the level of
ammonia present in the stored red blood cells are due to the production and release of ammonia
by the red blood cells. Moreover, the ammonia concentrations also increase as a result of
deamination of purines like adenine that are present in the storage solution as well as from the
vesiculation and formation of spherocytes. These spherocytic red blood cell populations are
rapidly removed from the circulation post transfusion (latt, Bawazir and Bruce 2014).
Figure 2: Graph showing distribution of potassium ion concentration of stored donor red
cell units obtained in supernatants. The graph shows the concentration of potassium ions
following 35 days of storage of red blood cells in refrigerators.
(Source: Flatt, Bawazir and Bruce 2014)
Accumulation of ammonia following storage of red blood cells
Apart from sodium ions and potassium ion concentrations that are affected following
storage of the red blood cells, the concentration of ammonia has also been found to increase in
the stored red blood cells. Normally, the concentration of ammonia found in the red blood cells
are more than that found in the plasma of the red blood cells. The increase in the level of
ammonia present in the stored red blood cells are due to the production and release of ammonia
by the red blood cells. Moreover, the ammonia concentrations also increase as a result of
deamination of purines like adenine that are present in the storage solution as well as from the
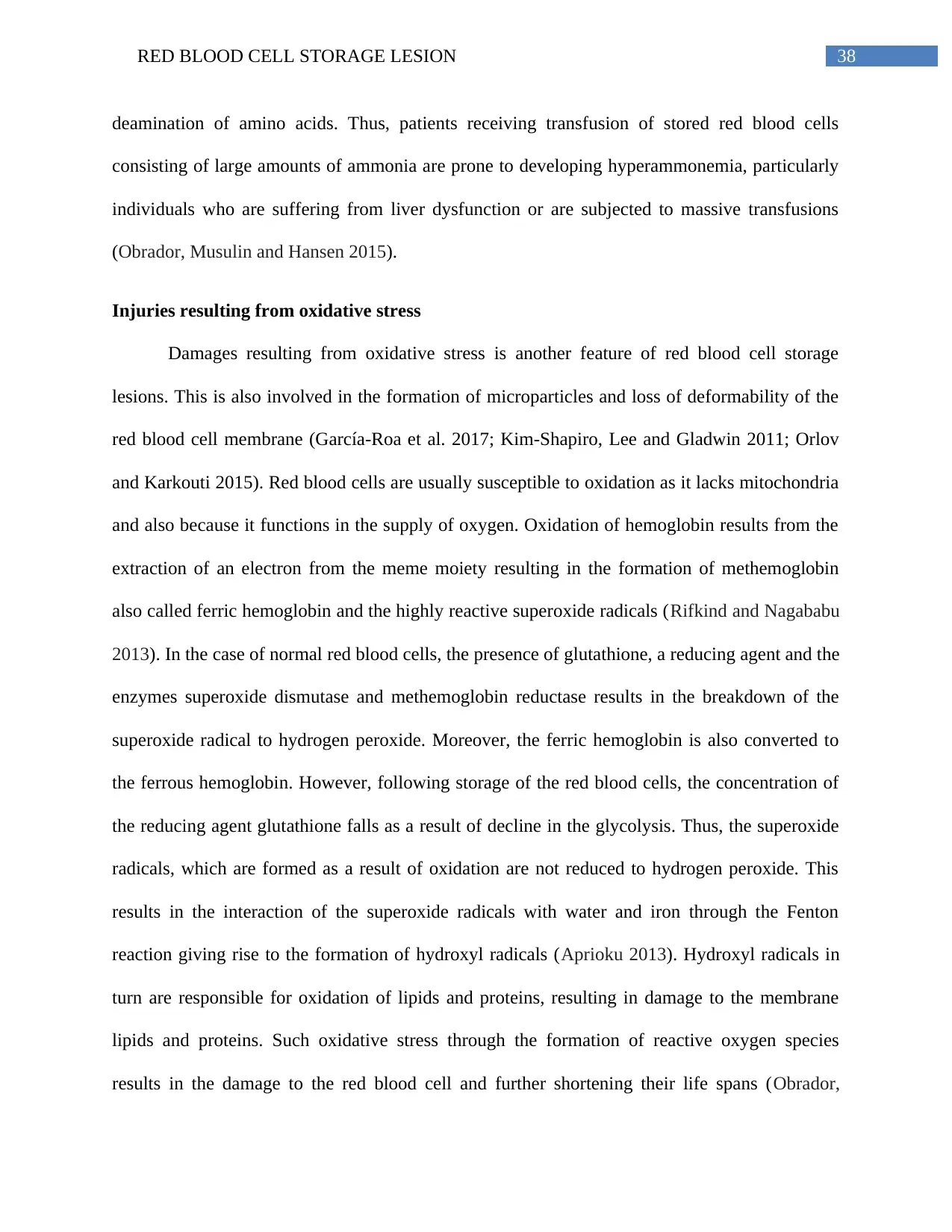
38RED BLOOD CELL STORAGE LESION
deamination of amino acids. Thus, patients receiving transfusion of stored red blood cells
consisting of large amounts of ammonia are prone to developing hyperammonemia, particularly
individuals who are suffering from liver dysfunction or are subjected to massive transfusions
(Obrador, Musulin and Hansen 2015).
Injuries resulting from oxidative stress
Damages resulting from oxidative stress is another feature of red blood cell storage
lesions. This is also involved in the formation of microparticles and loss of deformability of the
red blood cell membrane (García-Roa et al. 2017; Kim‐Shapiro, Lee and Gladwin 2011; Orlov
and Karkouti 2015). Red blood cells are usually susceptible to oxidation as it lacks mitochondria
and also because it functions in the supply of oxygen. Oxidation of hemoglobin results from the
extraction of an electron from the meme moiety resulting in the formation of methemoglobin
also called ferric hemoglobin and the highly reactive superoxide radicals (Rifkind and Nagababu
2013). In the case of normal red blood cells, the presence of glutathione, a reducing agent and the
enzymes superoxide dismutase and methemoglobin reductase results in the breakdown of the
superoxide radical to hydrogen peroxide. Moreover, the ferric hemoglobin is also converted to
the ferrous hemoglobin. However, following storage of the red blood cells, the concentration of
the reducing agent glutathione falls as a result of decline in the glycolysis. Thus, the superoxide
radicals, which are formed as a result of oxidation are not reduced to hydrogen peroxide. This
results in the interaction of the superoxide radicals with water and iron through the Fenton
reaction giving rise to the formation of hydroxyl radicals (Aprioku 2013). Hydroxyl radicals in
turn are responsible for oxidation of lipids and proteins, resulting in damage to the membrane
lipids and proteins. Such oxidative stress through the formation of reactive oxygen species
results in the damage to the red blood cell and further shortening their life spans (Obrador,
deamination of amino acids. Thus, patients receiving transfusion of stored red blood cells
consisting of large amounts of ammonia are prone to developing hyperammonemia, particularly
individuals who are suffering from liver dysfunction or are subjected to massive transfusions
(Obrador, Musulin and Hansen 2015).
Injuries resulting from oxidative stress
Damages resulting from oxidative stress is another feature of red blood cell storage
lesions. This is also involved in the formation of microparticles and loss of deformability of the
red blood cell membrane (García-Roa et al. 2017; Kim‐Shapiro, Lee and Gladwin 2011; Orlov
and Karkouti 2015). Red blood cells are usually susceptible to oxidation as it lacks mitochondria
and also because it functions in the supply of oxygen. Oxidation of hemoglobin results from the
extraction of an electron from the meme moiety resulting in the formation of methemoglobin
also called ferric hemoglobin and the highly reactive superoxide radicals (Rifkind and Nagababu
2013). In the case of normal red blood cells, the presence of glutathione, a reducing agent and the
enzymes superoxide dismutase and methemoglobin reductase results in the breakdown of the
superoxide radical to hydrogen peroxide. Moreover, the ferric hemoglobin is also converted to
the ferrous hemoglobin. However, following storage of the red blood cells, the concentration of
the reducing agent glutathione falls as a result of decline in the glycolysis. Thus, the superoxide
radicals, which are formed as a result of oxidation are not reduced to hydrogen peroxide. This
results in the interaction of the superoxide radicals with water and iron through the Fenton
reaction giving rise to the formation of hydroxyl radicals (Aprioku 2013). Hydroxyl radicals in
turn are responsible for oxidation of lipids and proteins, resulting in damage to the membrane
lipids and proteins. Such oxidative stress through the formation of reactive oxygen species
results in the damage to the red blood cell and further shortening their life spans (Obrador,
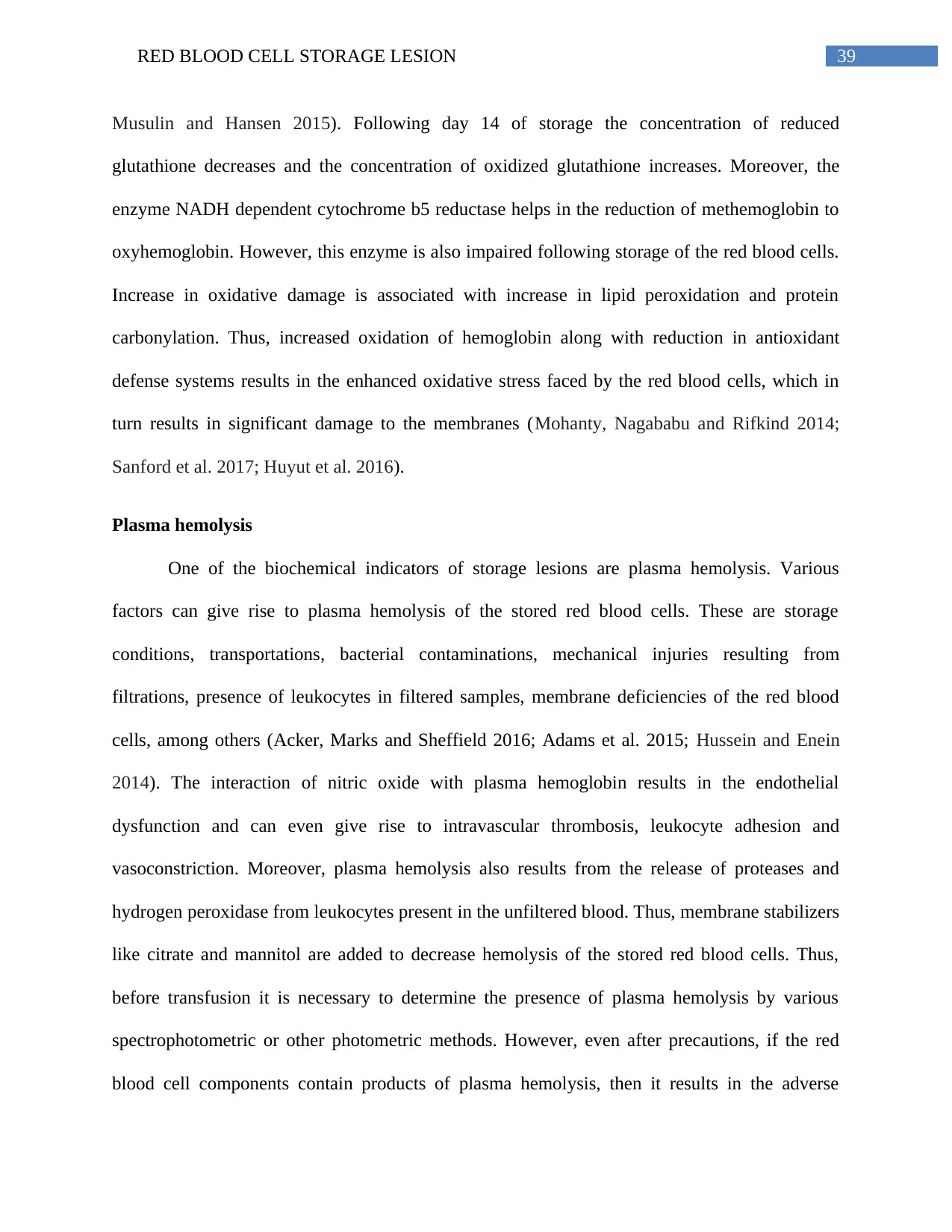
39RED BLOOD CELL STORAGE LESION
Musulin and Hansen 2015). Following day 14 of storage the concentration of reduced
glutathione decreases and the concentration of oxidized glutathione increases. Moreover, the
enzyme NADH dependent cytochrome b5 reductase helps in the reduction of methemoglobin to
oxyhemoglobin. However, this enzyme is also impaired following storage of the red blood cells.
Increase in oxidative damage is associated with increase in lipid peroxidation and protein
carbonylation. Thus, increased oxidation of hemoglobin along with reduction in antioxidant
defense systems results in the enhanced oxidative stress faced by the red blood cells, which in
turn results in significant damage to the membranes (Mohanty, Nagababu and Rifkind 2014;
Sanford et al. 2017; Huyut et al. 2016).
Plasma hemolysis
One of the biochemical indicators of storage lesions are plasma hemolysis. Various
factors can give rise to plasma hemolysis of the stored red blood cells. These are storage
conditions, transportations, bacterial contaminations, mechanical injuries resulting from
filtrations, presence of leukocytes in filtered samples, membrane deficiencies of the red blood
cells, among others (Acker, Marks and Sheffield 2016; Adams et al. 2015; Hussein and Enein
2014). The interaction of nitric oxide with plasma hemoglobin results in the endothelial
dysfunction and can even give rise to intravascular thrombosis, leukocyte adhesion and
vasoconstriction. Moreover, plasma hemolysis also results from the release of proteases and
hydrogen peroxidase from leukocytes present in the unfiltered blood. Thus, membrane stabilizers
like citrate and mannitol are added to decrease hemolysis of the stored red blood cells. Thus,
before transfusion it is necessary to determine the presence of plasma hemolysis by various
spectrophotometric or other photometric methods. However, even after precautions, if the red
blood cell components contain products of plasma hemolysis, then it results in the adverse
Musulin and Hansen 2015). Following day 14 of storage the concentration of reduced
glutathione decreases and the concentration of oxidized glutathione increases. Moreover, the
enzyme NADH dependent cytochrome b5 reductase helps in the reduction of methemoglobin to
oxyhemoglobin. However, this enzyme is also impaired following storage of the red blood cells.
Increase in oxidative damage is associated with increase in lipid peroxidation and protein
carbonylation. Thus, increased oxidation of hemoglobin along with reduction in antioxidant
defense systems results in the enhanced oxidative stress faced by the red blood cells, which in
turn results in significant damage to the membranes (Mohanty, Nagababu and Rifkind 2014;
Sanford et al. 2017; Huyut et al. 2016).
Plasma hemolysis
One of the biochemical indicators of storage lesions are plasma hemolysis. Various
factors can give rise to plasma hemolysis of the stored red blood cells. These are storage
conditions, transportations, bacterial contaminations, mechanical injuries resulting from
filtrations, presence of leukocytes in filtered samples, membrane deficiencies of the red blood
cells, among others (Acker, Marks and Sheffield 2016; Adams et al. 2015; Hussein and Enein
2014). The interaction of nitric oxide with plasma hemoglobin results in the endothelial
dysfunction and can even give rise to intravascular thrombosis, leukocyte adhesion and
vasoconstriction. Moreover, plasma hemolysis also results from the release of proteases and
hydrogen peroxidase from leukocytes present in the unfiltered blood. Thus, membrane stabilizers
like citrate and mannitol are added to decrease hemolysis of the stored red blood cells. Thus,
before transfusion it is necessary to determine the presence of plasma hemolysis by various
spectrophotometric or other photometric methods. However, even after precautions, if the red
blood cell components contain products of plasma hemolysis, then it results in the adverse
Secure Best Marks with AI Grader
Need help grading? Try our AI Grader for instant feedback on your assignments.
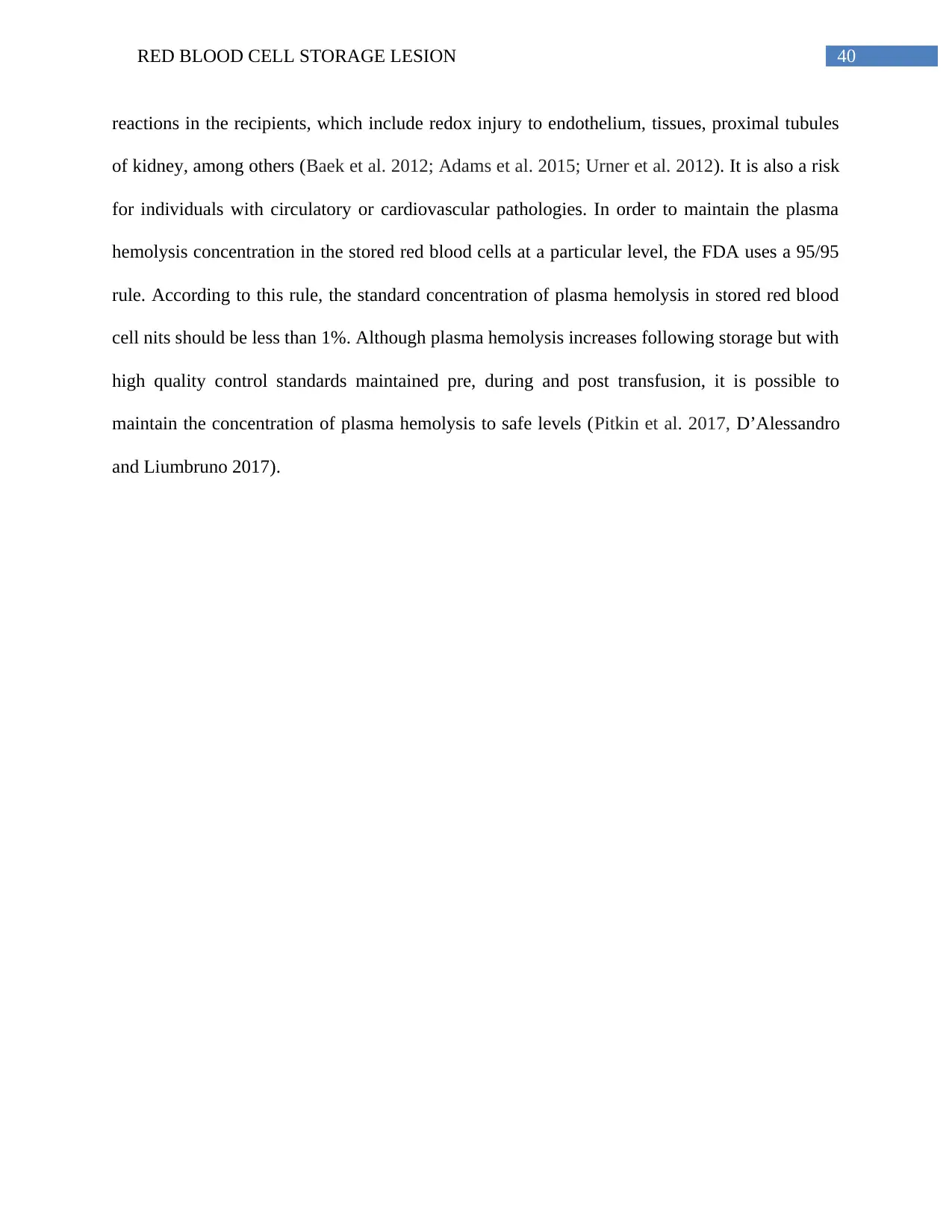
40RED BLOOD CELL STORAGE LESION
reactions in the recipients, which include redox injury to endothelium, tissues, proximal tubules
of kidney, among others (Baek et al. 2012; Adams et al. 2015; Urner et al. 2012). It is also a risk
for individuals with circulatory or cardiovascular pathologies. In order to maintain the plasma
hemolysis concentration in the stored red blood cells at a particular level, the FDA uses a 95/95
rule. According to this rule, the standard concentration of plasma hemolysis in stored red blood
cell nits should be less than 1%. Although plasma hemolysis increases following storage but with
high quality control standards maintained pre, during and post transfusion, it is possible to
maintain the concentration of plasma hemolysis to safe levels (Pitkin et al. 2017, D’Alessandro
and Liumbruno 2017).
reactions in the recipients, which include redox injury to endothelium, tissues, proximal tubules
of kidney, among others (Baek et al. 2012; Adams et al. 2015; Urner et al. 2012). It is also a risk
for individuals with circulatory or cardiovascular pathologies. In order to maintain the plasma
hemolysis concentration in the stored red blood cells at a particular level, the FDA uses a 95/95
rule. According to this rule, the standard concentration of plasma hemolysis in stored red blood
cell nits should be less than 1%. Although plasma hemolysis increases following storage but with
high quality control standards maintained pre, during and post transfusion, it is possible to
maintain the concentration of plasma hemolysis to safe levels (Pitkin et al. 2017, D’Alessandro
and Liumbruno 2017).
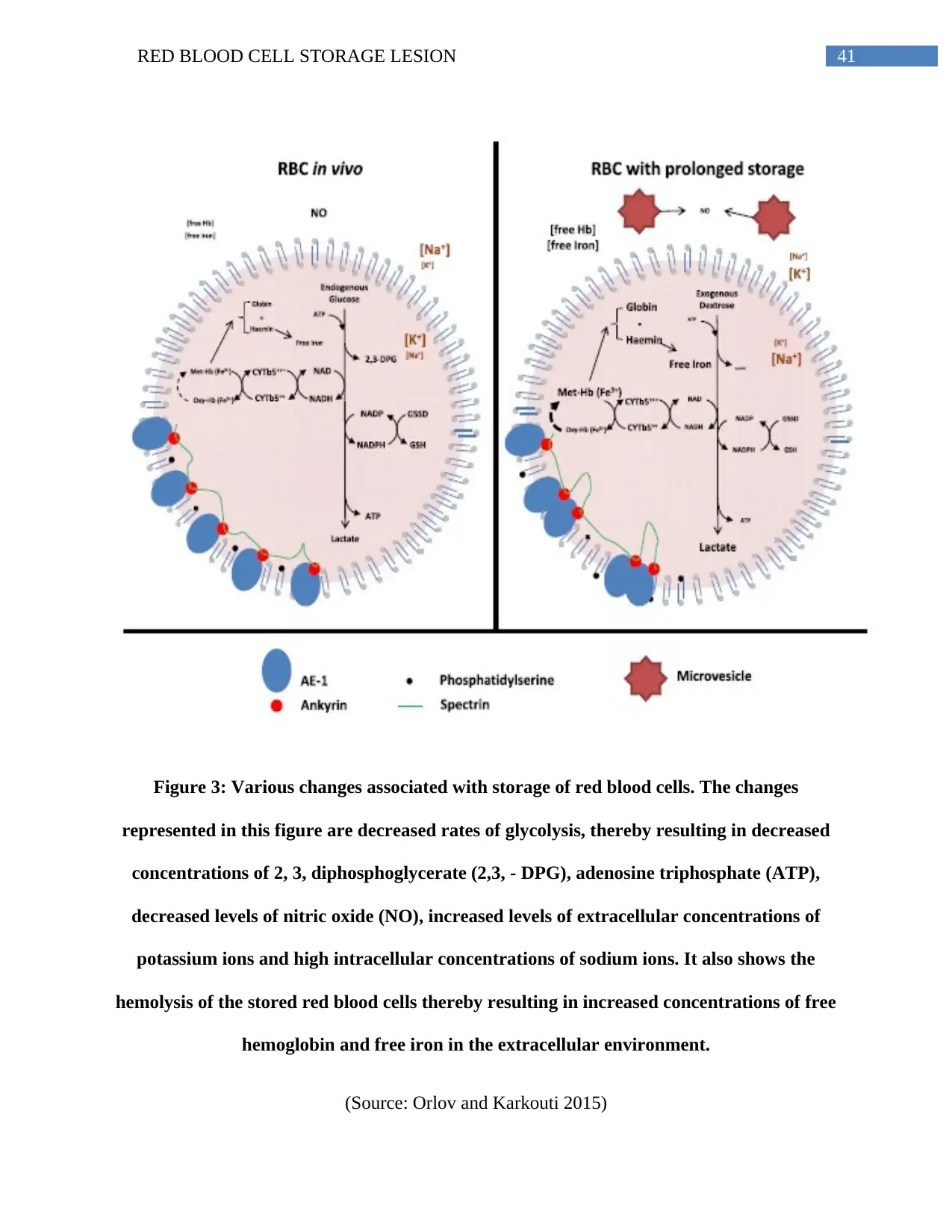
41RED BLOOD CELL STORAGE LESION
Figure 3: Various changes associated with storage of red blood cells. The changes
represented in this figure are decreased rates of glycolysis, thereby resulting in decreased
concentrations of 2, 3, diphosphoglycerate (2,3, - DPG), adenosine triphosphate (ATP),
decreased levels of nitric oxide (NO), increased levels of extracellular concentrations of
potassium ions and high intracellular concentrations of sodium ions. It also shows the
hemolysis of the stored red blood cells thereby resulting in increased concentrations of free
hemoglobin and free iron in the extracellular environment.
(Source: Orlov and Karkouti 2015)
Figure 3: Various changes associated with storage of red blood cells. The changes
represented in this figure are decreased rates of glycolysis, thereby resulting in decreased
concentrations of 2, 3, diphosphoglycerate (2,3, - DPG), adenosine triphosphate (ATP),
decreased levels of nitric oxide (NO), increased levels of extracellular concentrations of
potassium ions and high intracellular concentrations of sodium ions. It also shows the
hemolysis of the stored red blood cells thereby resulting in increased concentrations of free
hemoglobin and free iron in the extracellular environment.
(Source: Orlov and Karkouti 2015)
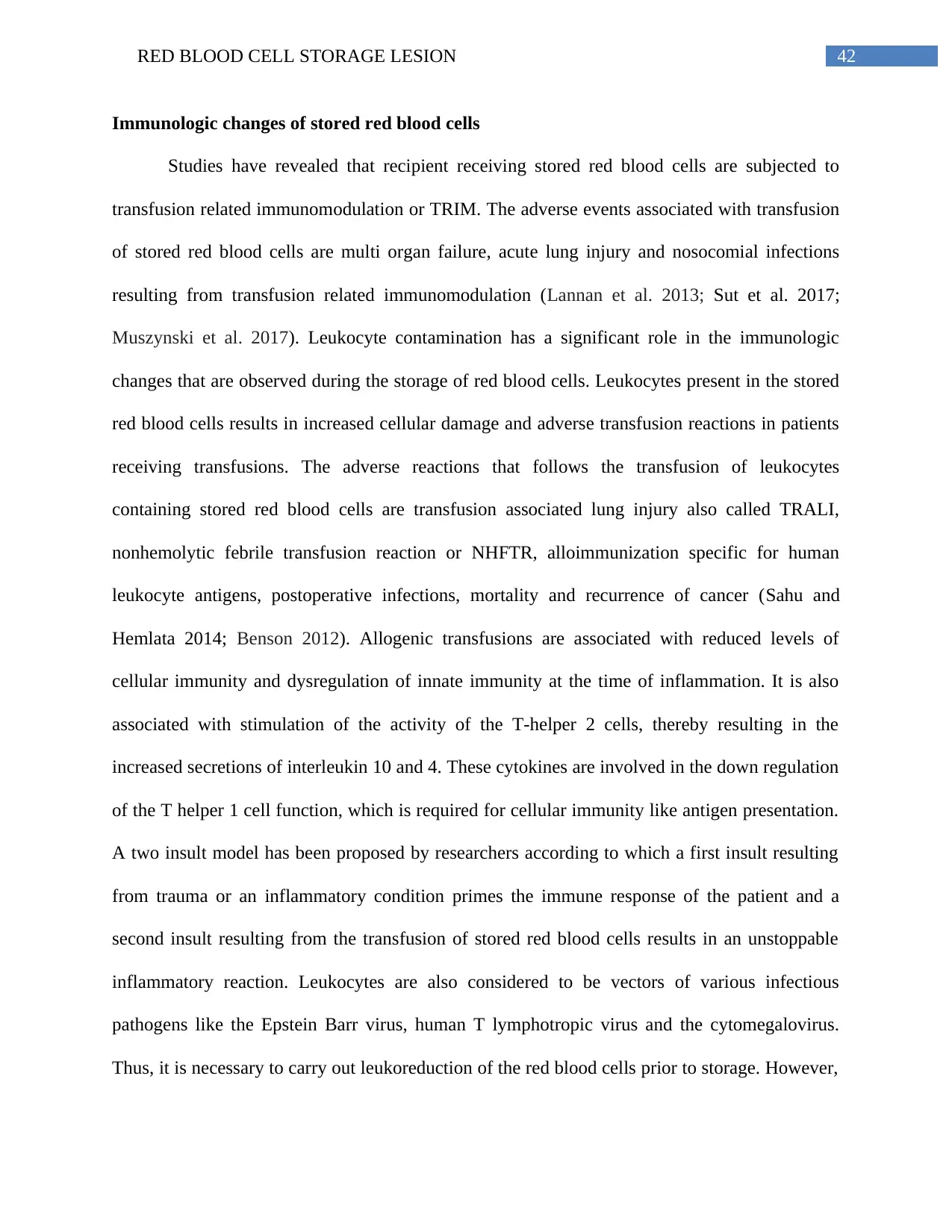
42RED BLOOD CELL STORAGE LESION
Immunologic changes of stored red blood cells
Studies have revealed that recipient receiving stored red blood cells are subjected to
transfusion related immunomodulation or TRIM. The adverse events associated with transfusion
of stored red blood cells are multi organ failure, acute lung injury and nosocomial infections
resulting from transfusion related immunomodulation (Lannan et al. 2013; Sut et al. 2017;
Muszynski et al. 2017). Leukocyte contamination has a significant role in the immunologic
changes that are observed during the storage of red blood cells. Leukocytes present in the stored
red blood cells results in increased cellular damage and adverse transfusion reactions in patients
receiving transfusions. The adverse reactions that follows the transfusion of leukocytes
containing stored red blood cells are transfusion associated lung injury also called TRALI,
nonhemolytic febrile transfusion reaction or NHFTR, alloimmunization specific for human
leukocyte antigens, postoperative infections, mortality and recurrence of cancer (Sahu and
Hemlata 2014; Benson 2012). Allogenic transfusions are associated with reduced levels of
cellular immunity and dysregulation of innate immunity at the time of inflammation. It is also
associated with stimulation of the activity of the T-helper 2 cells, thereby resulting in the
increased secretions of interleukin 10 and 4. These cytokines are involved in the down regulation
of the T helper 1 cell function, which is required for cellular immunity like antigen presentation.
A two insult model has been proposed by researchers according to which a first insult resulting
from trauma or an inflammatory condition primes the immune response of the patient and a
second insult resulting from the transfusion of stored red blood cells results in an unstoppable
inflammatory reaction. Leukocytes are also considered to be vectors of various infectious
pathogens like the Epstein Barr virus, human T lymphotropic virus and the cytomegalovirus.
Thus, it is necessary to carry out leukoreduction of the red blood cells prior to storage. However,
Immunologic changes of stored red blood cells
Studies have revealed that recipient receiving stored red blood cells are subjected to
transfusion related immunomodulation or TRIM. The adverse events associated with transfusion
of stored red blood cells are multi organ failure, acute lung injury and nosocomial infections
resulting from transfusion related immunomodulation (Lannan et al. 2013; Sut et al. 2017;
Muszynski et al. 2017). Leukocyte contamination has a significant role in the immunologic
changes that are observed during the storage of red blood cells. Leukocytes present in the stored
red blood cells results in increased cellular damage and adverse transfusion reactions in patients
receiving transfusions. The adverse reactions that follows the transfusion of leukocytes
containing stored red blood cells are transfusion associated lung injury also called TRALI,
nonhemolytic febrile transfusion reaction or NHFTR, alloimmunization specific for human
leukocyte antigens, postoperative infections, mortality and recurrence of cancer (Sahu and
Hemlata 2014; Benson 2012). Allogenic transfusions are associated with reduced levels of
cellular immunity and dysregulation of innate immunity at the time of inflammation. It is also
associated with stimulation of the activity of the T-helper 2 cells, thereby resulting in the
increased secretions of interleukin 10 and 4. These cytokines are involved in the down regulation
of the T helper 1 cell function, which is required for cellular immunity like antigen presentation.
A two insult model has been proposed by researchers according to which a first insult resulting
from trauma or an inflammatory condition primes the immune response of the patient and a
second insult resulting from the transfusion of stored red blood cells results in an unstoppable
inflammatory reaction. Leukocytes are also considered to be vectors of various infectious
pathogens like the Epstein Barr virus, human T lymphotropic virus and the cytomegalovirus.
Thus, it is necessary to carry out leukoreduction of the red blood cells prior to storage. However,
Paraphrase This Document
Need a fresh take? Get an instant paraphrase of this document with our AI Paraphraser
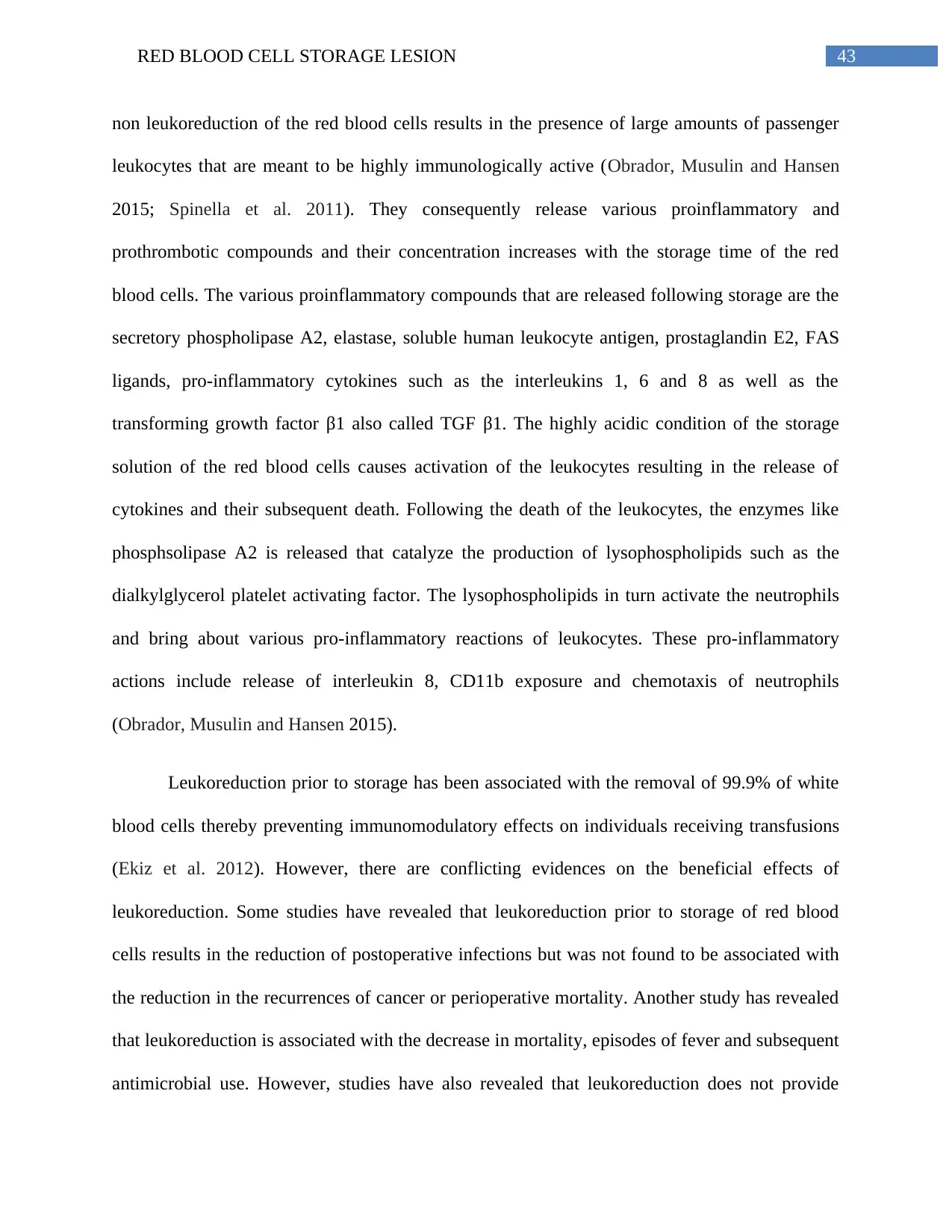
43RED BLOOD CELL STORAGE LESION
non leukoreduction of the red blood cells results in the presence of large amounts of passenger
leukocytes that are meant to be highly immunologically active (Obrador, Musulin and Hansen
2015; Spinella et al. 2011). They consequently release various proinflammatory and
prothrombotic compounds and their concentration increases with the storage time of the red
blood cells. The various proinflammatory compounds that are released following storage are the
secretory phospholipase A2, elastase, soluble human leukocyte antigen, prostaglandin E2, FAS
ligands, pro-inflammatory cytokines such as the interleukins 1, 6 and 8 as well as the
transforming growth factor β1 also called TGF β1. The highly acidic condition of the storage
solution of the red blood cells causes activation of the leukocytes resulting in the release of
cytokines and their subsequent death. Following the death of the leukocytes, the enzymes like
phosphsolipase A2 is released that catalyze the production of lysophospholipids such as the
dialkylglycerol platelet activating factor. The lysophospholipids in turn activate the neutrophils
and bring about various pro-inflammatory reactions of leukocytes. These pro-inflammatory
actions include release of interleukin 8, CD11b exposure and chemotaxis of neutrophils
(Obrador, Musulin and Hansen 2015).
Leukoreduction prior to storage has been associated with the removal of 99.9% of white
blood cells thereby preventing immunomodulatory effects on individuals receiving transfusions
(Ekiz et al. 2012). However, there are conflicting evidences on the beneficial effects of
leukoreduction. Some studies have revealed that leukoreduction prior to storage of red blood
cells results in the reduction of postoperative infections but was not found to be associated with
the reduction in the recurrences of cancer or perioperative mortality. Another study has revealed
that leukoreduction is associated with the decrease in mortality, episodes of fever and subsequent
antimicrobial use. However, studies have also revealed that leukoreduction does not provide
non leukoreduction of the red blood cells results in the presence of large amounts of passenger
leukocytes that are meant to be highly immunologically active (Obrador, Musulin and Hansen
2015; Spinella et al. 2011). They consequently release various proinflammatory and
prothrombotic compounds and their concentration increases with the storage time of the red
blood cells. The various proinflammatory compounds that are released following storage are the
secretory phospholipase A2, elastase, soluble human leukocyte antigen, prostaglandin E2, FAS
ligands, pro-inflammatory cytokines such as the interleukins 1, 6 and 8 as well as the
transforming growth factor β1 also called TGF β1. The highly acidic condition of the storage
solution of the red blood cells causes activation of the leukocytes resulting in the release of
cytokines and their subsequent death. Following the death of the leukocytes, the enzymes like
phosphsolipase A2 is released that catalyze the production of lysophospholipids such as the
dialkylglycerol platelet activating factor. The lysophospholipids in turn activate the neutrophils
and bring about various pro-inflammatory reactions of leukocytes. These pro-inflammatory
actions include release of interleukin 8, CD11b exposure and chemotaxis of neutrophils
(Obrador, Musulin and Hansen 2015).
Leukoreduction prior to storage has been associated with the removal of 99.9% of white
blood cells thereby preventing immunomodulatory effects on individuals receiving transfusions
(Ekiz et al. 2012). However, there are conflicting evidences on the beneficial effects of
leukoreduction. Some studies have revealed that leukoreduction prior to storage of red blood
cells results in the reduction of postoperative infections but was not found to be associated with
the reduction in the recurrences of cancer or perioperative mortality. Another study has revealed
that leukoreduction is associated with the decrease in mortality, episodes of fever and subsequent
antimicrobial use. However, studies have also revealed that leukoreduction does not provide
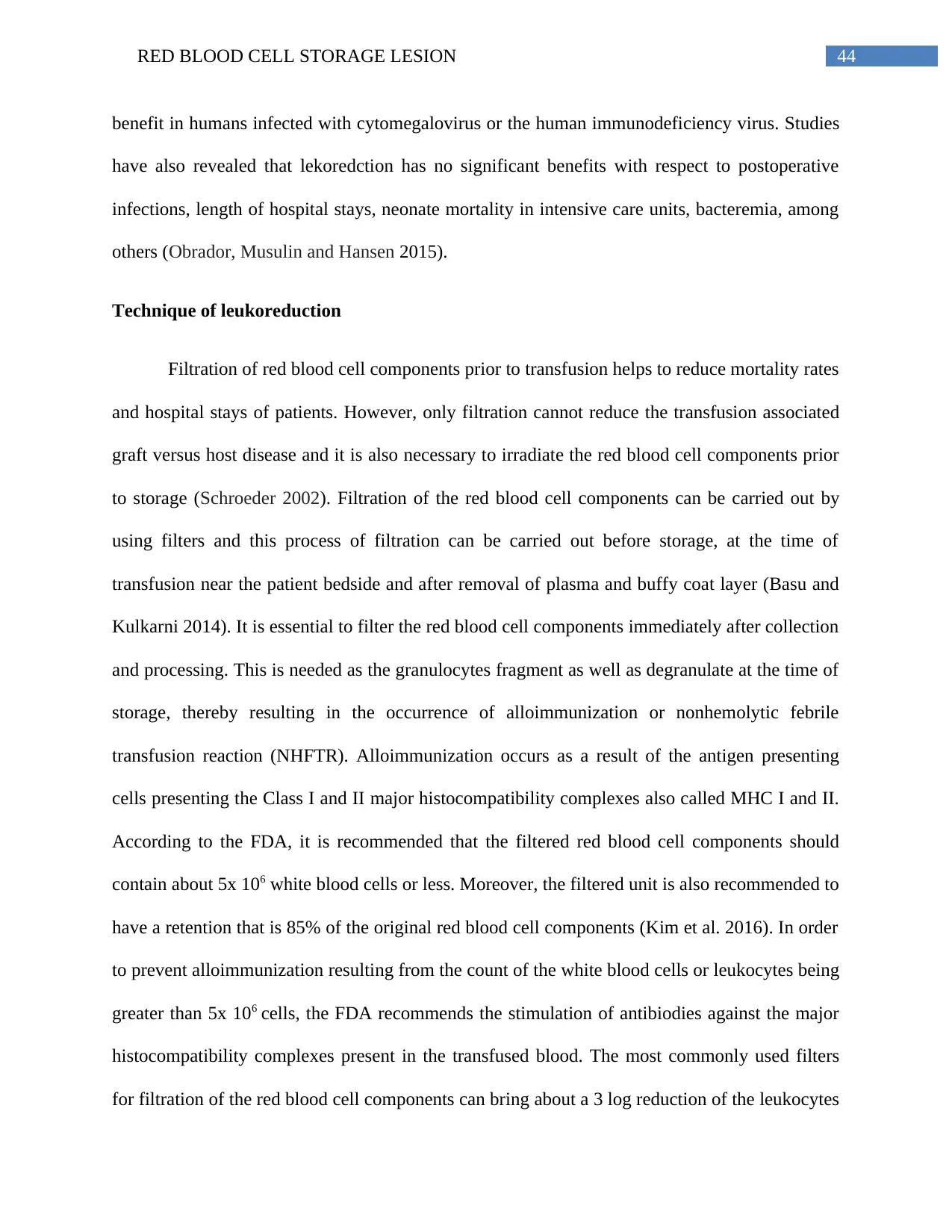
44RED BLOOD CELL STORAGE LESION
benefit in humans infected with cytomegalovirus or the human immunodeficiency virus. Studies
have also revealed that lekoredction has no significant benefits with respect to postoperative
infections, length of hospital stays, neonate mortality in intensive care units, bacteremia, among
others (Obrador, Musulin and Hansen 2015).
Technique of leukoreduction
Filtration of red blood cell components prior to transfusion helps to reduce mortality rates
and hospital stays of patients. However, only filtration cannot reduce the transfusion associated
graft versus host disease and it is also necessary to irradiate the red blood cell components prior
to storage (Schroeder 2002). Filtration of the red blood cell components can be carried out by
using filters and this process of filtration can be carried out before storage, at the time of
transfusion near the patient bedside and after removal of plasma and buffy coat layer (Basu and
Kulkarni 2014). It is essential to filter the red blood cell components immediately after collection
and processing. This is needed as the granulocytes fragment as well as degranulate at the time of
storage, thereby resulting in the occurrence of alloimmunization or nonhemolytic febrile
transfusion reaction (NHFTR). Alloimmunization occurs as a result of the antigen presenting
cells presenting the Class I and II major histocompatibility complexes also called MHC I and II.
According to the FDA, it is recommended that the filtered red blood cell components should
contain about 5x 106 white blood cells or less. Moreover, the filtered unit is also recommended to
have a retention that is 85% of the original red blood cell components (Kim et al. 2016). In order
to prevent alloimmunization resulting from the count of the white blood cells or leukocytes being
greater than 5x 106 cells, the FDA recommends the stimulation of antibiodies against the major
histocompatibility complexes present in the transfused blood. The most commonly used filters
for filtration of the red blood cell components can bring about a 3 log reduction of the leukocytes
benefit in humans infected with cytomegalovirus or the human immunodeficiency virus. Studies
have also revealed that lekoredction has no significant benefits with respect to postoperative
infections, length of hospital stays, neonate mortality in intensive care units, bacteremia, among
others (Obrador, Musulin and Hansen 2015).
Technique of leukoreduction
Filtration of red blood cell components prior to transfusion helps to reduce mortality rates
and hospital stays of patients. However, only filtration cannot reduce the transfusion associated
graft versus host disease and it is also necessary to irradiate the red blood cell components prior
to storage (Schroeder 2002). Filtration of the red blood cell components can be carried out by
using filters and this process of filtration can be carried out before storage, at the time of
transfusion near the patient bedside and after removal of plasma and buffy coat layer (Basu and
Kulkarni 2014). It is essential to filter the red blood cell components immediately after collection
and processing. This is needed as the granulocytes fragment as well as degranulate at the time of
storage, thereby resulting in the occurrence of alloimmunization or nonhemolytic febrile
transfusion reaction (NHFTR). Alloimmunization occurs as a result of the antigen presenting
cells presenting the Class I and II major histocompatibility complexes also called MHC I and II.
According to the FDA, it is recommended that the filtered red blood cell components should
contain about 5x 106 white blood cells or less. Moreover, the filtered unit is also recommended to
have a retention that is 85% of the original red blood cell components (Kim et al. 2016). In order
to prevent alloimmunization resulting from the count of the white blood cells or leukocytes being
greater than 5x 106 cells, the FDA recommends the stimulation of antibiodies against the major
histocompatibility complexes present in the transfused blood. The most commonly used filters
for filtration of the red blood cell components can bring about a 3 log reduction of the leukocytes
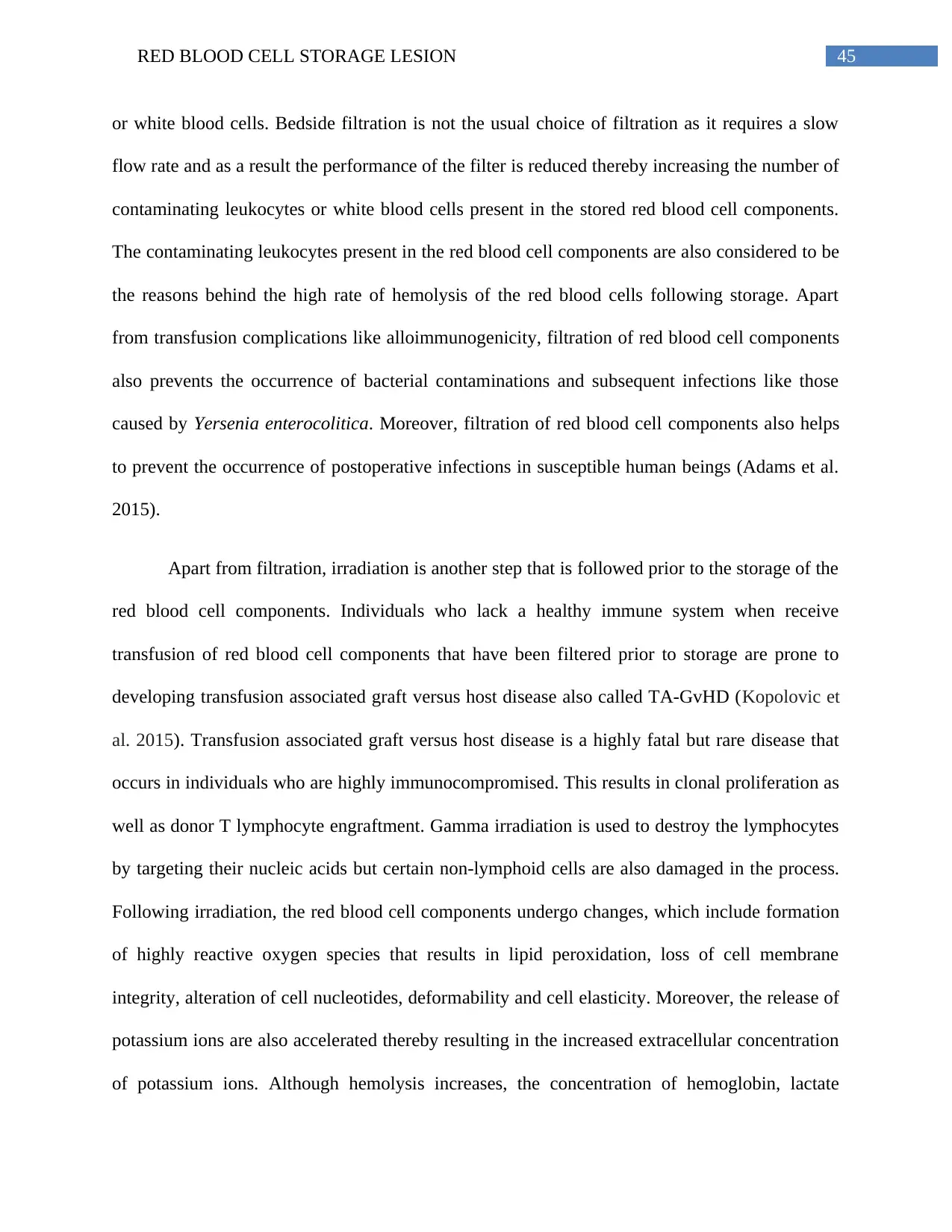
45RED BLOOD CELL STORAGE LESION
or white blood cells. Bedside filtration is not the usual choice of filtration as it requires a slow
flow rate and as a result the performance of the filter is reduced thereby increasing the number of
contaminating leukocytes or white blood cells present in the stored red blood cell components.
The contaminating leukocytes present in the red blood cell components are also considered to be
the reasons behind the high rate of hemolysis of the red blood cells following storage. Apart
from transfusion complications like alloimmunogenicity, filtration of red blood cell components
also prevents the occurrence of bacterial contaminations and subsequent infections like those
caused by Yersenia enterocolitica. Moreover, filtration of red blood cell components also helps
to prevent the occurrence of postoperative infections in susceptible human beings (Adams et al.
2015).
Apart from filtration, irradiation is another step that is followed prior to the storage of the
red blood cell components. Individuals who lack a healthy immune system when receive
transfusion of red blood cell components that have been filtered prior to storage are prone to
developing transfusion associated graft versus host disease also called TA-GvHD (Kopolovic et
al. 2015). Transfusion associated graft versus host disease is a highly fatal but rare disease that
occurs in individuals who are highly immunocompromised. This results in clonal proliferation as
well as donor T lymphocyte engraftment. Gamma irradiation is used to destroy the lymphocytes
by targeting their nucleic acids but certain non-lymphoid cells are also damaged in the process.
Following irradiation, the red blood cell components undergo changes, which include formation
of highly reactive oxygen species that results in lipid peroxidation, loss of cell membrane
integrity, alteration of cell nucleotides, deformability and cell elasticity. Moreover, the release of
potassium ions are also accelerated thereby resulting in the increased extracellular concentration
of potassium ions. Although hemolysis increases, the concentration of hemoglobin, lactate
or white blood cells. Bedside filtration is not the usual choice of filtration as it requires a slow
flow rate and as a result the performance of the filter is reduced thereby increasing the number of
contaminating leukocytes or white blood cells present in the stored red blood cell components.
The contaminating leukocytes present in the red blood cell components are also considered to be
the reasons behind the high rate of hemolysis of the red blood cells following storage. Apart
from transfusion complications like alloimmunogenicity, filtration of red blood cell components
also prevents the occurrence of bacterial contaminations and subsequent infections like those
caused by Yersenia enterocolitica. Moreover, filtration of red blood cell components also helps
to prevent the occurrence of postoperative infections in susceptible human beings (Adams et al.
2015).
Apart from filtration, irradiation is another step that is followed prior to the storage of the
red blood cell components. Individuals who lack a healthy immune system when receive
transfusion of red blood cell components that have been filtered prior to storage are prone to
developing transfusion associated graft versus host disease also called TA-GvHD (Kopolovic et
al. 2015). Transfusion associated graft versus host disease is a highly fatal but rare disease that
occurs in individuals who are highly immunocompromised. This results in clonal proliferation as
well as donor T lymphocyte engraftment. Gamma irradiation is used to destroy the lymphocytes
by targeting their nucleic acids but certain non-lymphoid cells are also damaged in the process.
Following irradiation, the red blood cell components undergo changes, which include formation
of highly reactive oxygen species that results in lipid peroxidation, loss of cell membrane
integrity, alteration of cell nucleotides, deformability and cell elasticity. Moreover, the release of
potassium ions are also accelerated thereby resulting in the increased extracellular concentration
of potassium ions. Although hemolysis increases, the concentration of hemoglobin, lactate
Secure Best Marks with AI Grader
Need help grading? Try our AI Grader for instant feedback on your assignments.
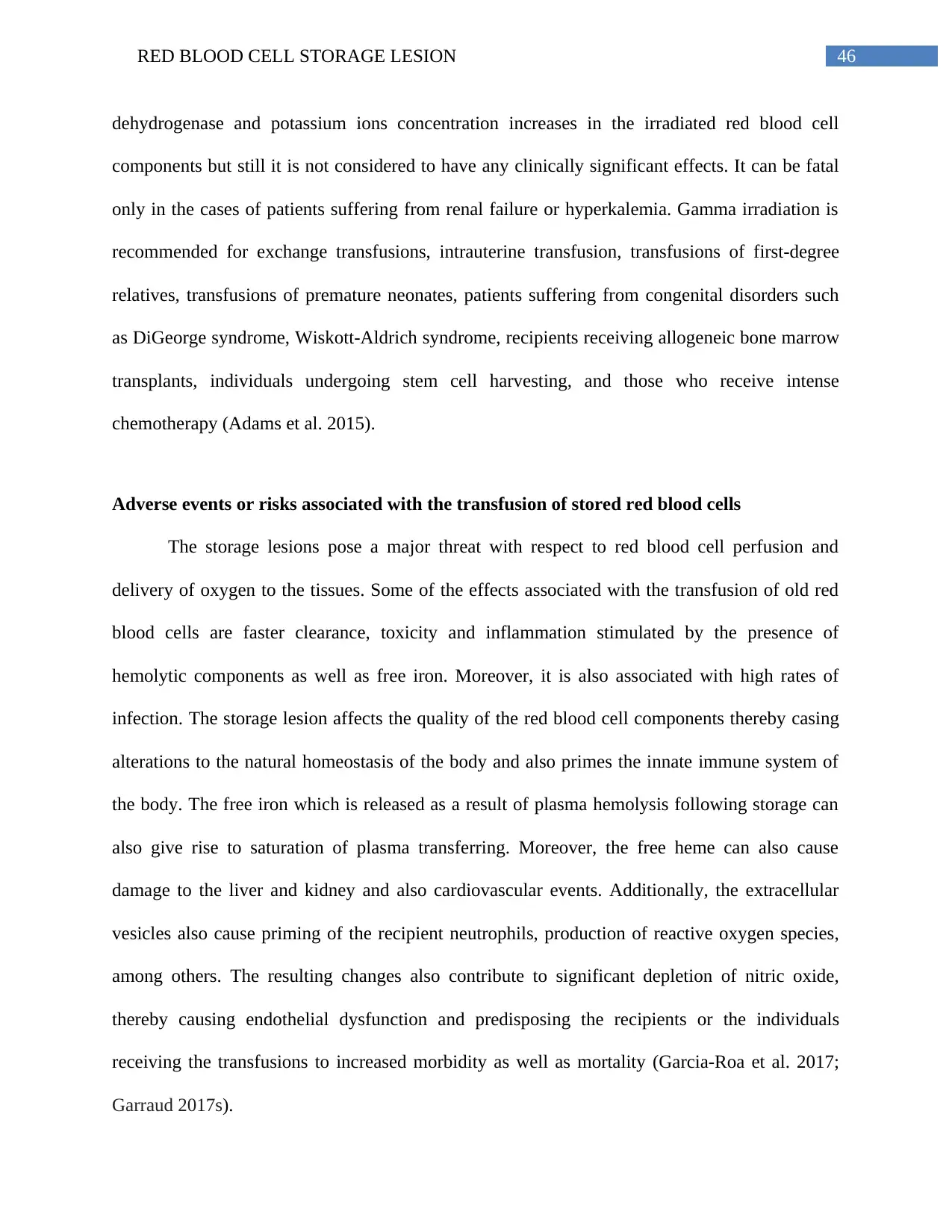
46RED BLOOD CELL STORAGE LESION
dehydrogenase and potassium ions concentration increases in the irradiated red blood cell
components but still it is not considered to have any clinically significant effects. It can be fatal
only in the cases of patients suffering from renal failure or hyperkalemia. Gamma irradiation is
recommended for exchange transfusions, intrauterine transfusion, transfusions of first-degree
relatives, transfusions of premature neonates, patients suffering from congenital disorders such
as DiGeorge syndrome, Wiskott-Aldrich syndrome, recipients receiving allogeneic bone marrow
transplants, individuals undergoing stem cell harvesting, and those who receive intense
chemotherapy (Adams et al. 2015).
Adverse events or risks associated with the transfusion of stored red blood cells
The storage lesions pose a major threat with respect to red blood cell perfusion and
delivery of oxygen to the tissues. Some of the effects associated with the transfusion of old red
blood cells are faster clearance, toxicity and inflammation stimulated by the presence of
hemolytic components as well as free iron. Moreover, it is also associated with high rates of
infection. The storage lesion affects the quality of the red blood cell components thereby casing
alterations to the natural homeostasis of the body and also primes the innate immune system of
the body. The free iron which is released as a result of plasma hemolysis following storage can
also give rise to saturation of plasma transferring. Moreover, the free heme can also cause
damage to the liver and kidney and also cardiovascular events. Additionally, the extracellular
vesicles also cause priming of the recipient neutrophils, production of reactive oxygen species,
among others. The resulting changes also contribute to significant depletion of nitric oxide,
thereby causing endothelial dysfunction and predisposing the recipients or the individuals
receiving the transfusions to increased morbidity as well as mortality (Garcia-Roa et al. 2017;
Garraud 2017s).
dehydrogenase and potassium ions concentration increases in the irradiated red blood cell
components but still it is not considered to have any clinically significant effects. It can be fatal
only in the cases of patients suffering from renal failure or hyperkalemia. Gamma irradiation is
recommended for exchange transfusions, intrauterine transfusion, transfusions of first-degree
relatives, transfusions of premature neonates, patients suffering from congenital disorders such
as DiGeorge syndrome, Wiskott-Aldrich syndrome, recipients receiving allogeneic bone marrow
transplants, individuals undergoing stem cell harvesting, and those who receive intense
chemotherapy (Adams et al. 2015).
Adverse events or risks associated with the transfusion of stored red blood cells
The storage lesions pose a major threat with respect to red blood cell perfusion and
delivery of oxygen to the tissues. Some of the effects associated with the transfusion of old red
blood cells are faster clearance, toxicity and inflammation stimulated by the presence of
hemolytic components as well as free iron. Moreover, it is also associated with high rates of
infection. The storage lesion affects the quality of the red blood cell components thereby casing
alterations to the natural homeostasis of the body and also primes the innate immune system of
the body. The free iron which is released as a result of plasma hemolysis following storage can
also give rise to saturation of plasma transferring. Moreover, the free heme can also cause
damage to the liver and kidney and also cardiovascular events. Additionally, the extracellular
vesicles also cause priming of the recipient neutrophils, production of reactive oxygen species,
among others. The resulting changes also contribute to significant depletion of nitric oxide,
thereby causing endothelial dysfunction and predisposing the recipients or the individuals
receiving the transfusions to increased morbidity as well as mortality (Garcia-Roa et al. 2017;
Garraud 2017s).
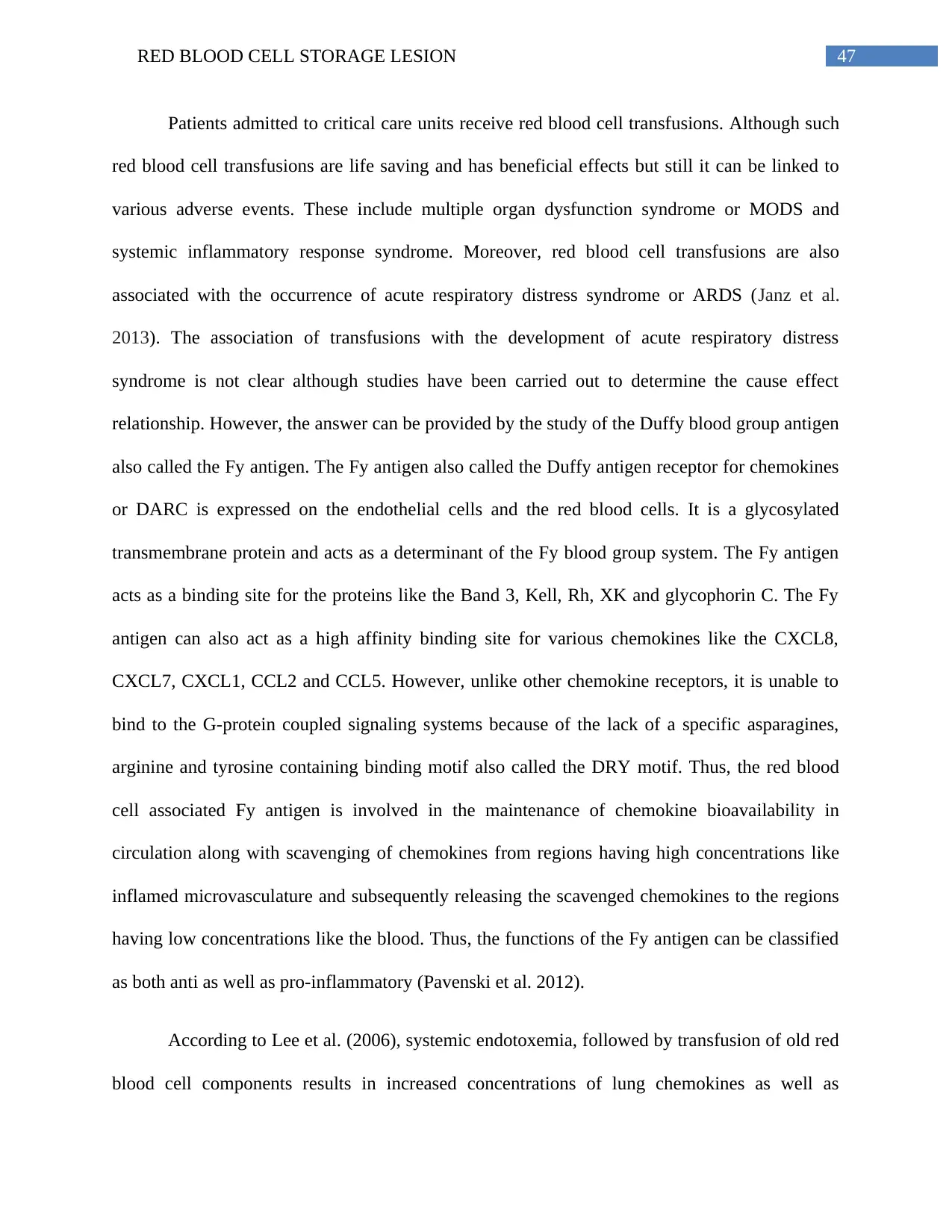
47RED BLOOD CELL STORAGE LESION
Patients admitted to critical care units receive red blood cell transfusions. Although such
red blood cell transfusions are life saving and has beneficial effects but still it can be linked to
various adverse events. These include multiple organ dysfunction syndrome or MODS and
systemic inflammatory response syndrome. Moreover, red blood cell transfusions are also
associated with the occurrence of acute respiratory distress syndrome or ARDS (Janz et al.
2013). The association of transfusions with the development of acute respiratory distress
syndrome is not clear although studies have been carried out to determine the cause effect
relationship. However, the answer can be provided by the study of the Duffy blood group antigen
also called the Fy antigen. The Fy antigen also called the Duffy antigen receptor for chemokines
or DARC is expressed on the endothelial cells and the red blood cells. It is a glycosylated
transmembrane protein and acts as a determinant of the Fy blood group system. The Fy antigen
acts as a binding site for the proteins like the Band 3, Kell, Rh, XK and glycophorin C. The Fy
antigen can also act as a high affinity binding site for various chemokines like the CXCL8,
CXCL7, CXCL1, CCL2 and CCL5. However, unlike other chemokine receptors, it is unable to
bind to the G-protein coupled signaling systems because of the lack of a specific asparagines,
arginine and tyrosine containing binding motif also called the DRY motif. Thus, the red blood
cell associated Fy antigen is involved in the maintenance of chemokine bioavailability in
circulation along with scavenging of chemokines from regions having high concentrations like
inflamed microvasculature and subsequently releasing the scavenged chemokines to the regions
having low concentrations like the blood. Thus, the functions of the Fy antigen can be classified
as both anti as well as pro-inflammatory (Pavenski et al. 2012).
According to Lee et al. (2006), systemic endotoxemia, followed by transfusion of old red
blood cell components results in increased concentrations of lung chemokines as well as
Patients admitted to critical care units receive red blood cell transfusions. Although such
red blood cell transfusions are life saving and has beneficial effects but still it can be linked to
various adverse events. These include multiple organ dysfunction syndrome or MODS and
systemic inflammatory response syndrome. Moreover, red blood cell transfusions are also
associated with the occurrence of acute respiratory distress syndrome or ARDS (Janz et al.
2013). The association of transfusions with the development of acute respiratory distress
syndrome is not clear although studies have been carried out to determine the cause effect
relationship. However, the answer can be provided by the study of the Duffy blood group antigen
also called the Fy antigen. The Fy antigen also called the Duffy antigen receptor for chemokines
or DARC is expressed on the endothelial cells and the red blood cells. It is a glycosylated
transmembrane protein and acts as a determinant of the Fy blood group system. The Fy antigen
acts as a binding site for the proteins like the Band 3, Kell, Rh, XK and glycophorin C. The Fy
antigen can also act as a high affinity binding site for various chemokines like the CXCL8,
CXCL7, CXCL1, CCL2 and CCL5. However, unlike other chemokine receptors, it is unable to
bind to the G-protein coupled signaling systems because of the lack of a specific asparagines,
arginine and tyrosine containing binding motif also called the DRY motif. Thus, the red blood
cell associated Fy antigen is involved in the maintenance of chemokine bioavailability in
circulation along with scavenging of chemokines from regions having high concentrations like
inflamed microvasculature and subsequently releasing the scavenged chemokines to the regions
having low concentrations like the blood. Thus, the functions of the Fy antigen can be classified
as both anti as well as pro-inflammatory (Pavenski et al. 2012).
According to Lee et al. (2006), systemic endotoxemia, followed by transfusion of old red
blood cell components results in increased concentrations of lung chemokines as well as
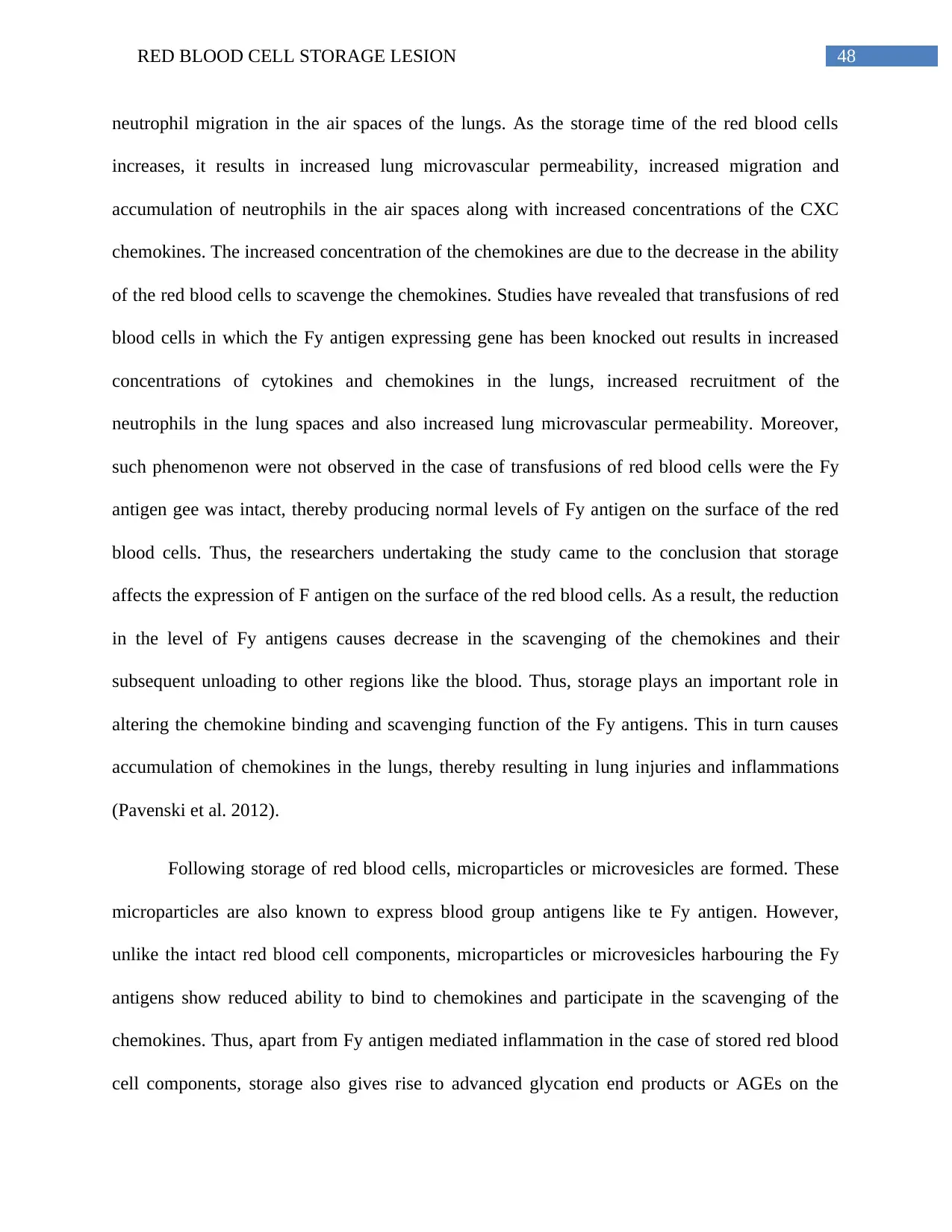
48RED BLOOD CELL STORAGE LESION
neutrophil migration in the air spaces of the lungs. As the storage time of the red blood cells
increases, it results in increased lung microvascular permeability, increased migration and
accumulation of neutrophils in the air spaces along with increased concentrations of the CXC
chemokines. The increased concentration of the chemokines are due to the decrease in the ability
of the red blood cells to scavenge the chemokines. Studies have revealed that transfusions of red
blood cells in which the Fy antigen expressing gene has been knocked out results in increased
concentrations of cytokines and chemokines in the lungs, increased recruitment of the
neutrophils in the lung spaces and also increased lung microvascular permeability. Moreover,
such phenomenon were not observed in the case of transfusions of red blood cells were the Fy
antigen gee was intact, thereby producing normal levels of Fy antigen on the surface of the red
blood cells. Thus, the researchers undertaking the study came to the conclusion that storage
affects the expression of F antigen on the surface of the red blood cells. As a result, the reduction
in the level of Fy antigens causes decrease in the scavenging of the chemokines and their
subsequent unloading to other regions like the blood. Thus, storage plays an important role in
altering the chemokine binding and scavenging function of the Fy antigens. This in turn causes
accumulation of chemokines in the lungs, thereby resulting in lung injuries and inflammations
(Pavenski et al. 2012).
Following storage of red blood cells, microparticles or microvesicles are formed. These
microparticles are also known to express blood group antigens like te Fy antigen. However,
unlike the intact red blood cell components, microparticles or microvesicles harbouring the Fy
antigens show reduced ability to bind to chemokines and participate in the scavenging of the
chemokines. Thus, apart from Fy antigen mediated inflammation in the case of stored red blood
cell components, storage also gives rise to advanced glycation end products or AGEs on the
neutrophil migration in the air spaces of the lungs. As the storage time of the red blood cells
increases, it results in increased lung microvascular permeability, increased migration and
accumulation of neutrophils in the air spaces along with increased concentrations of the CXC
chemokines. The increased concentration of the chemokines are due to the decrease in the ability
of the red blood cells to scavenge the chemokines. Studies have revealed that transfusions of red
blood cells in which the Fy antigen expressing gene has been knocked out results in increased
concentrations of cytokines and chemokines in the lungs, increased recruitment of the
neutrophils in the lung spaces and also increased lung microvascular permeability. Moreover,
such phenomenon were not observed in the case of transfusions of red blood cells were the Fy
antigen gee was intact, thereby producing normal levels of Fy antigen on the surface of the red
blood cells. Thus, the researchers undertaking the study came to the conclusion that storage
affects the expression of F antigen on the surface of the red blood cells. As a result, the reduction
in the level of Fy antigens causes decrease in the scavenging of the chemokines and their
subsequent unloading to other regions like the blood. Thus, storage plays an important role in
altering the chemokine binding and scavenging function of the Fy antigens. This in turn causes
accumulation of chemokines in the lungs, thereby resulting in lung injuries and inflammations
(Pavenski et al. 2012).
Following storage of red blood cells, microparticles or microvesicles are formed. These
microparticles are also known to express blood group antigens like te Fy antigen. However,
unlike the intact red blood cell components, microparticles or microvesicles harbouring the Fy
antigens show reduced ability to bind to chemokines and participate in the scavenging of the
chemokines. Thus, apart from Fy antigen mediated inflammation in the case of stored red blood
cell components, storage also gives rise to advanced glycation end products or AGEs on the
Paraphrase This Document
Need a fresh take? Get an instant paraphrase of this document with our AI Paraphraser
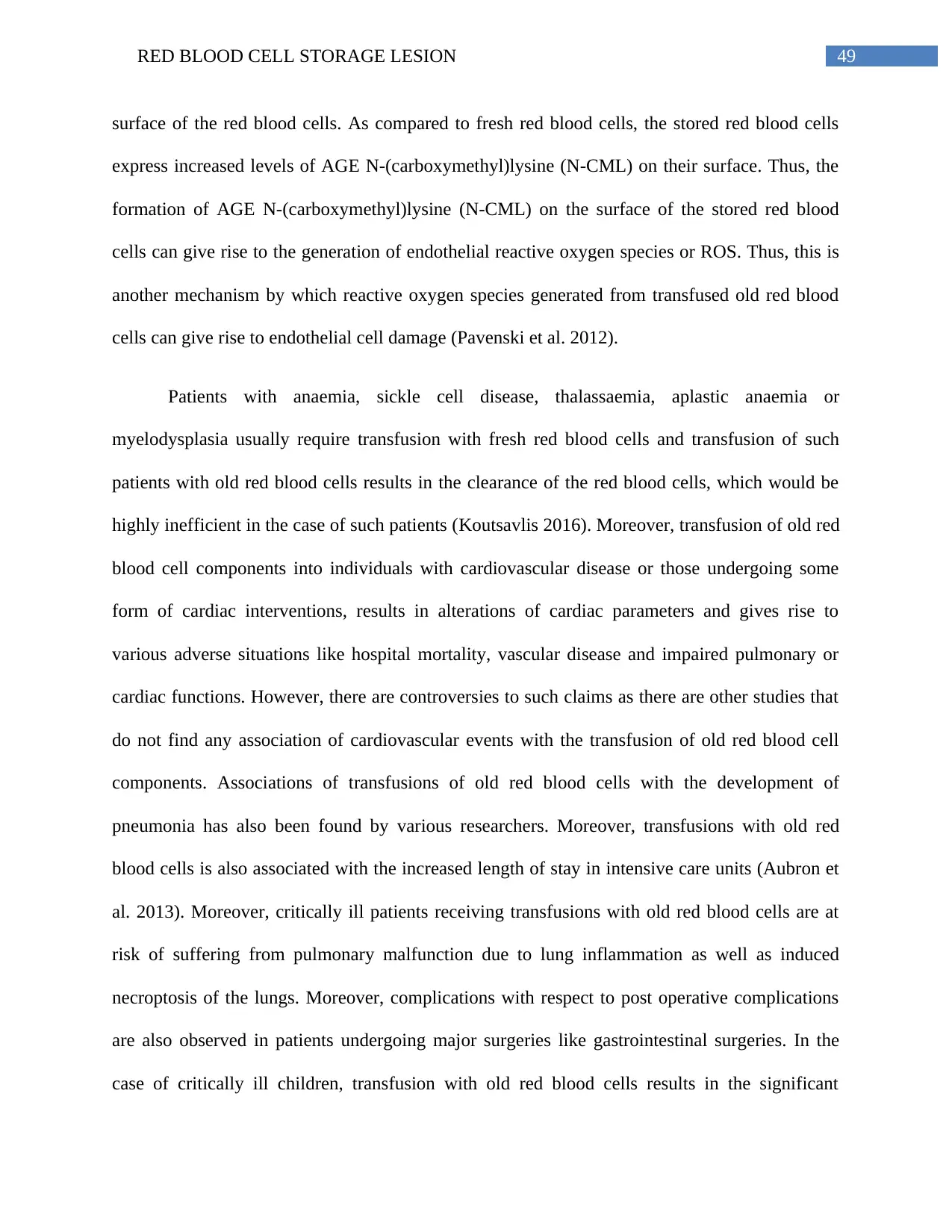
49RED BLOOD CELL STORAGE LESION
surface of the red blood cells. As compared to fresh red blood cells, the stored red blood cells
express increased levels of AGE N-(carboxymethyl)lysine (N-CML) on their surface. Thus, the
formation of AGE N-(carboxymethyl)lysine (N-CML) on the surface of the stored red blood
cells can give rise to the generation of endothelial reactive oxygen species or ROS. Thus, this is
another mechanism by which reactive oxygen species generated from transfused old red blood
cells can give rise to endothelial cell damage (Pavenski et al. 2012).
Patients with anaemia, sickle cell disease, thalassaemia, aplastic anaemia or
myelodysplasia usually require transfusion with fresh red blood cells and transfusion of such
patients with old red blood cells results in the clearance of the red blood cells, which would be
highly inefficient in the case of such patients (Koutsavlis 2016). Moreover, transfusion of old red
blood cell components into individuals with cardiovascular disease or those undergoing some
form of cardiac interventions, results in alterations of cardiac parameters and gives rise to
various adverse situations like hospital mortality, vascular disease and impaired pulmonary or
cardiac functions. However, there are controversies to such claims as there are other studies that
do not find any association of cardiovascular events with the transfusion of old red blood cell
components. Associations of transfusions of old red blood cells with the development of
pneumonia has also been found by various researchers. Moreover, transfusions with old red
blood cells is also associated with the increased length of stay in intensive care units (Aubron et
al. 2013). Moreover, critically ill patients receiving transfusions with old red blood cells are at
risk of suffering from pulmonary malfunction due to lung inflammation as well as induced
necroptosis of the lungs. Moreover, complications with respect to post operative complications
are also observed in patients undergoing major surgeries like gastrointestinal surgeries. In the
case of critically ill children, transfusion with old red blood cells results in the significant
surface of the red blood cells. As compared to fresh red blood cells, the stored red blood cells
express increased levels of AGE N-(carboxymethyl)lysine (N-CML) on their surface. Thus, the
formation of AGE N-(carboxymethyl)lysine (N-CML) on the surface of the stored red blood
cells can give rise to the generation of endothelial reactive oxygen species or ROS. Thus, this is
another mechanism by which reactive oxygen species generated from transfused old red blood
cells can give rise to endothelial cell damage (Pavenski et al. 2012).
Patients with anaemia, sickle cell disease, thalassaemia, aplastic anaemia or
myelodysplasia usually require transfusion with fresh red blood cells and transfusion of such
patients with old red blood cells results in the clearance of the red blood cells, which would be
highly inefficient in the case of such patients (Koutsavlis 2016). Moreover, transfusion of old red
blood cell components into individuals with cardiovascular disease or those undergoing some
form of cardiac interventions, results in alterations of cardiac parameters and gives rise to
various adverse situations like hospital mortality, vascular disease and impaired pulmonary or
cardiac functions. However, there are controversies to such claims as there are other studies that
do not find any association of cardiovascular events with the transfusion of old red blood cell
components. Associations of transfusions of old red blood cells with the development of
pneumonia has also been found by various researchers. Moreover, transfusions with old red
blood cells is also associated with the increased length of stay in intensive care units (Aubron et
al. 2013). Moreover, critically ill patients receiving transfusions with old red blood cells are at
risk of suffering from pulmonary malfunction due to lung inflammation as well as induced
necroptosis of the lungs. Moreover, complications with respect to post operative complications
are also observed in patients undergoing major surgeries like gastrointestinal surgeries. In the
case of critically ill children, transfusion with old red blood cells results in the significant
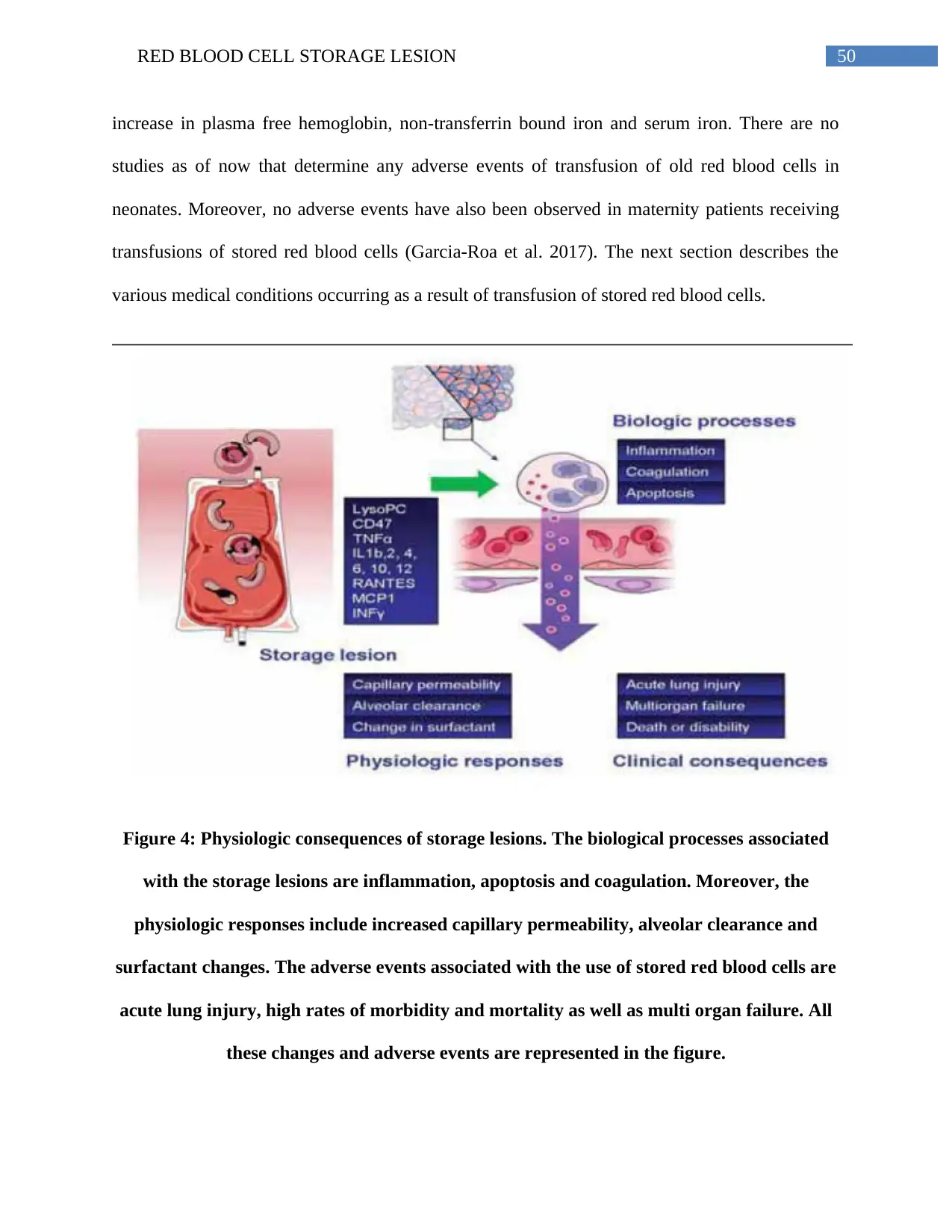
50RED BLOOD CELL STORAGE LESION
increase in plasma free hemoglobin, non-transferrin bound iron and serum iron. There are no
studies as of now that determine any adverse events of transfusion of old red blood cells in
neonates. Moreover, no adverse events have also been observed in maternity patients receiving
transfusions of stored red blood cells (Garcia-Roa et al. 2017). The next section describes the
various medical conditions occurring as a result of transfusion of stored red blood cells.
Figure 4: Physiologic consequences of storage lesions. The biological processes associated
with the storage lesions are inflammation, apoptosis and coagulation. Moreover, the
physiologic responses include increased capillary permeability, alveolar clearance and
surfactant changes. The adverse events associated with the use of stored red blood cells are
acute lung injury, high rates of morbidity and mortality as well as multi organ failure. All
these changes and adverse events are represented in the figure.
increase in plasma free hemoglobin, non-transferrin bound iron and serum iron. There are no
studies as of now that determine any adverse events of transfusion of old red blood cells in
neonates. Moreover, no adverse events have also been observed in maternity patients receiving
transfusions of stored red blood cells (Garcia-Roa et al. 2017). The next section describes the
various medical conditions occurring as a result of transfusion of stored red blood cells.
Figure 4: Physiologic consequences of storage lesions. The biological processes associated
with the storage lesions are inflammation, apoptosis and coagulation. Moreover, the
physiologic responses include increased capillary permeability, alveolar clearance and
surfactant changes. The adverse events associated with the use of stored red blood cells are
acute lung injury, high rates of morbidity and mortality as well as multi organ failure. All
these changes and adverse events are represented in the figure.
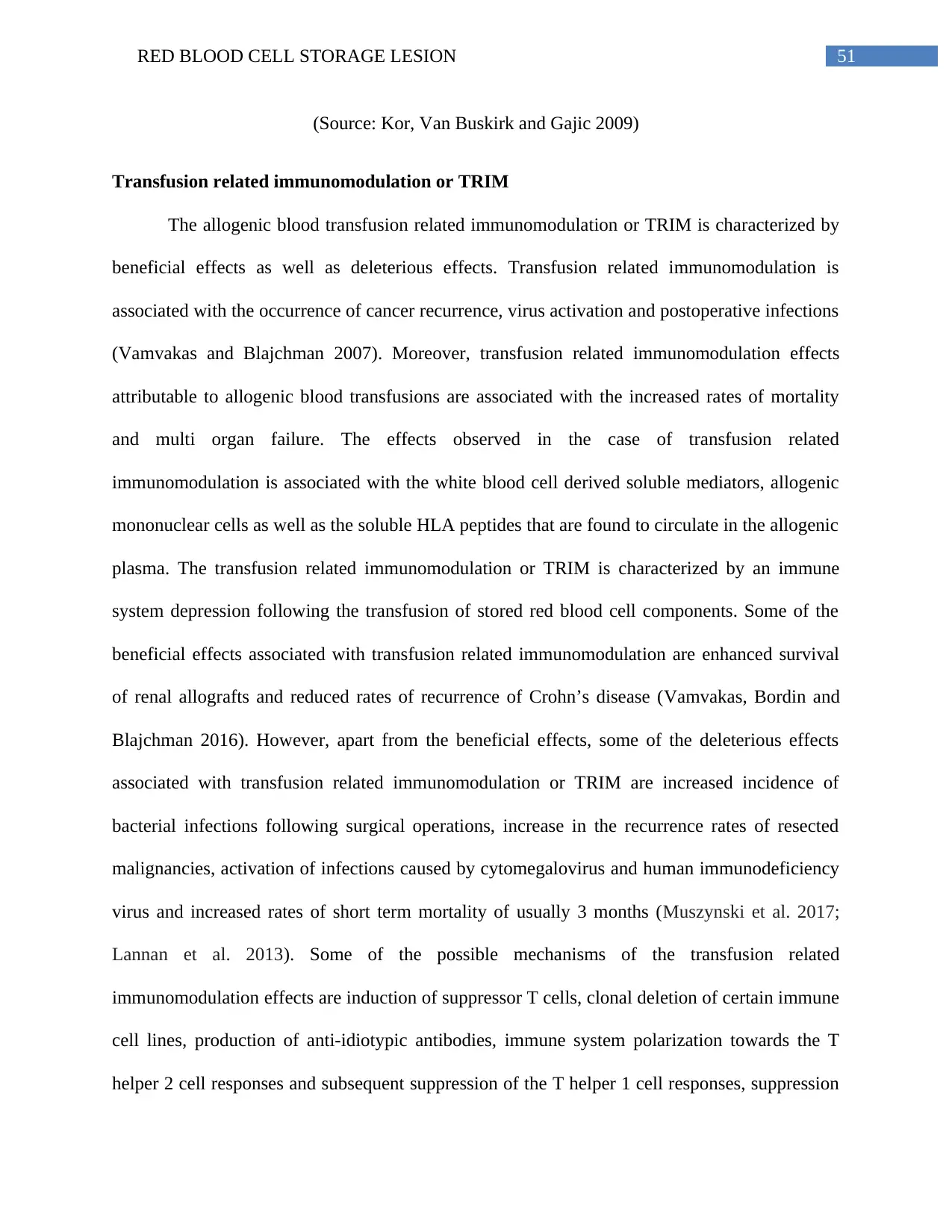
51RED BLOOD CELL STORAGE LESION
(Source: Kor, Van Buskirk and Gajic 2009)
Transfusion related immunomodulation or TRIM
The allogenic blood transfusion related immunomodulation or TRIM is characterized by
beneficial effects as well as deleterious effects. Transfusion related immunomodulation is
associated with the occurrence of cancer recurrence, virus activation and postoperative infections
(Vamvakas and Blajchman 2007). Moreover, transfusion related immunomodulation effects
attributable to allogenic blood transfusions are associated with the increased rates of mortality
and multi organ failure. The effects observed in the case of transfusion related
immunomodulation is associated with the white blood cell derived soluble mediators, allogenic
mononuclear cells as well as the soluble HLA peptides that are found to circulate in the allogenic
plasma. The transfusion related immunomodulation or TRIM is characterized by an immune
system depression following the transfusion of stored red blood cell components. Some of the
beneficial effects associated with transfusion related immunomodulation are enhanced survival
of renal allografts and reduced rates of recurrence of Crohn’s disease (Vamvakas, Bordin and
Blajchman 2016). However, apart from the beneficial effects, some of the deleterious effects
associated with transfusion related immunomodulation or TRIM are increased incidence of
bacterial infections following surgical operations, increase in the recurrence rates of resected
malignancies, activation of infections caused by cytomegalovirus and human immunodeficiency
virus and increased rates of short term mortality of usually 3 months (Muszynski et al. 2017;
Lannan et al. 2013). Some of the possible mechanisms of the transfusion related
immunomodulation effects are induction of suppressor T cells, clonal deletion of certain immune
cell lines, production of anti-idiotypic antibodies, immune system polarization towards the T
helper 2 cell responses and subsequent suppression of the T helper 1 cell responses, suppression
(Source: Kor, Van Buskirk and Gajic 2009)
Transfusion related immunomodulation or TRIM
The allogenic blood transfusion related immunomodulation or TRIM is characterized by
beneficial effects as well as deleterious effects. Transfusion related immunomodulation is
associated with the occurrence of cancer recurrence, virus activation and postoperative infections
(Vamvakas and Blajchman 2007). Moreover, transfusion related immunomodulation effects
attributable to allogenic blood transfusions are associated with the increased rates of mortality
and multi organ failure. The effects observed in the case of transfusion related
immunomodulation is associated with the white blood cell derived soluble mediators, allogenic
mononuclear cells as well as the soluble HLA peptides that are found to circulate in the allogenic
plasma. The transfusion related immunomodulation or TRIM is characterized by an immune
system depression following the transfusion of stored red blood cell components. Some of the
beneficial effects associated with transfusion related immunomodulation are enhanced survival
of renal allografts and reduced rates of recurrence of Crohn’s disease (Vamvakas, Bordin and
Blajchman 2016). However, apart from the beneficial effects, some of the deleterious effects
associated with transfusion related immunomodulation or TRIM are increased incidence of
bacterial infections following surgical operations, increase in the recurrence rates of resected
malignancies, activation of infections caused by cytomegalovirus and human immunodeficiency
virus and increased rates of short term mortality of usually 3 months (Muszynski et al. 2017;
Lannan et al. 2013). Some of the possible mechanisms of the transfusion related
immunomodulation effects are induction of suppressor T cells, clonal deletion of certain immune
cell lines, production of anti-idiotypic antibodies, immune system polarization towards the T
helper 2 cell responses and subsequent suppression of the T helper 1 cell responses, suppression
Secure Best Marks with AI Grader
Need help grading? Try our AI Grader for instant feedback on your assignments.
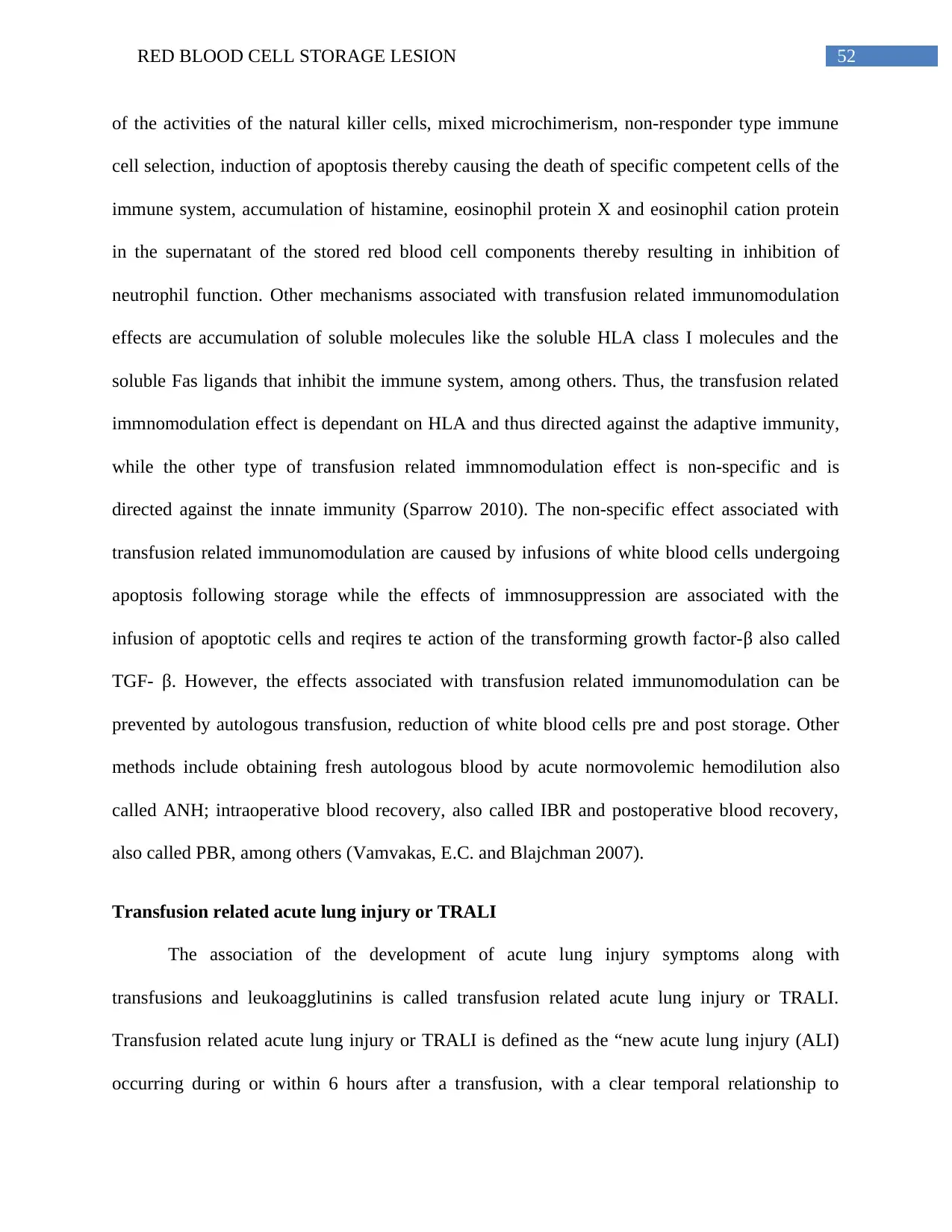
52RED BLOOD CELL STORAGE LESION
of the activities of the natural killer cells, mixed microchimerism, non-responder type immune
cell selection, induction of apoptosis thereby causing the death of specific competent cells of the
immune system, accumulation of histamine, eosinophil protein X and eosinophil cation protein
in the supernatant of the stored red blood cell components thereby resulting in inhibition of
neutrophil function. Other mechanisms associated with transfusion related immunomodulation
effects are accumulation of soluble molecules like the soluble HLA class I molecules and the
soluble Fas ligands that inhibit the immune system, among others. Thus, the transfusion related
immnomodulation effect is dependant on HLA and thus directed against the adaptive immunity,
while the other type of transfusion related immnomodulation effect is non-specific and is
directed against the innate immunity (Sparrow 2010). The non-specific effect associated with
transfusion related immunomodulation are caused by infusions of white blood cells undergoing
apoptosis following storage while the effects of immnosuppression are associated with the
infusion of apoptotic cells and reqires te action of the transforming growth factor-β also called
TGF- β. However, the effects associated with transfusion related immunomodulation can be
prevented by autologous transfusion, reduction of white blood cells pre and post storage. Other
methods include obtaining fresh autologous blood by acute normovolemic hemodilution also
called ANH; intraoperative blood recovery, also called IBR and postoperative blood recovery,
also called PBR, among others (Vamvakas, E.C. and Blajchman 2007).
Transfusion related acute lung injury or TRALI
The association of the development of acute lung injury symptoms along with
transfusions and leukoagglutinins is called transfusion related acute lung injury or TRALI.
Transfusion related acute lung injury or TRALI is defined as the “new acute lung injury (ALI)
occurring during or within 6 hours after a transfusion, with a clear temporal relationship to
of the activities of the natural killer cells, mixed microchimerism, non-responder type immune
cell selection, induction of apoptosis thereby causing the death of specific competent cells of the
immune system, accumulation of histamine, eosinophil protein X and eosinophil cation protein
in the supernatant of the stored red blood cell components thereby resulting in inhibition of
neutrophil function. Other mechanisms associated with transfusion related immunomodulation
effects are accumulation of soluble molecules like the soluble HLA class I molecules and the
soluble Fas ligands that inhibit the immune system, among others. Thus, the transfusion related
immnomodulation effect is dependant on HLA and thus directed against the adaptive immunity,
while the other type of transfusion related immnomodulation effect is non-specific and is
directed against the innate immunity (Sparrow 2010). The non-specific effect associated with
transfusion related immunomodulation are caused by infusions of white blood cells undergoing
apoptosis following storage while the effects of immnosuppression are associated with the
infusion of apoptotic cells and reqires te action of the transforming growth factor-β also called
TGF- β. However, the effects associated with transfusion related immunomodulation can be
prevented by autologous transfusion, reduction of white blood cells pre and post storage. Other
methods include obtaining fresh autologous blood by acute normovolemic hemodilution also
called ANH; intraoperative blood recovery, also called IBR and postoperative blood recovery,
also called PBR, among others (Vamvakas, E.C. and Blajchman 2007).
Transfusion related acute lung injury or TRALI
The association of the development of acute lung injury symptoms along with
transfusions and leukoagglutinins is called transfusion related acute lung injury or TRALI.
Transfusion related acute lung injury or TRALI is defined as the “new acute lung injury (ALI)
occurring during or within 6 hours after a transfusion, with a clear temporal relationship to
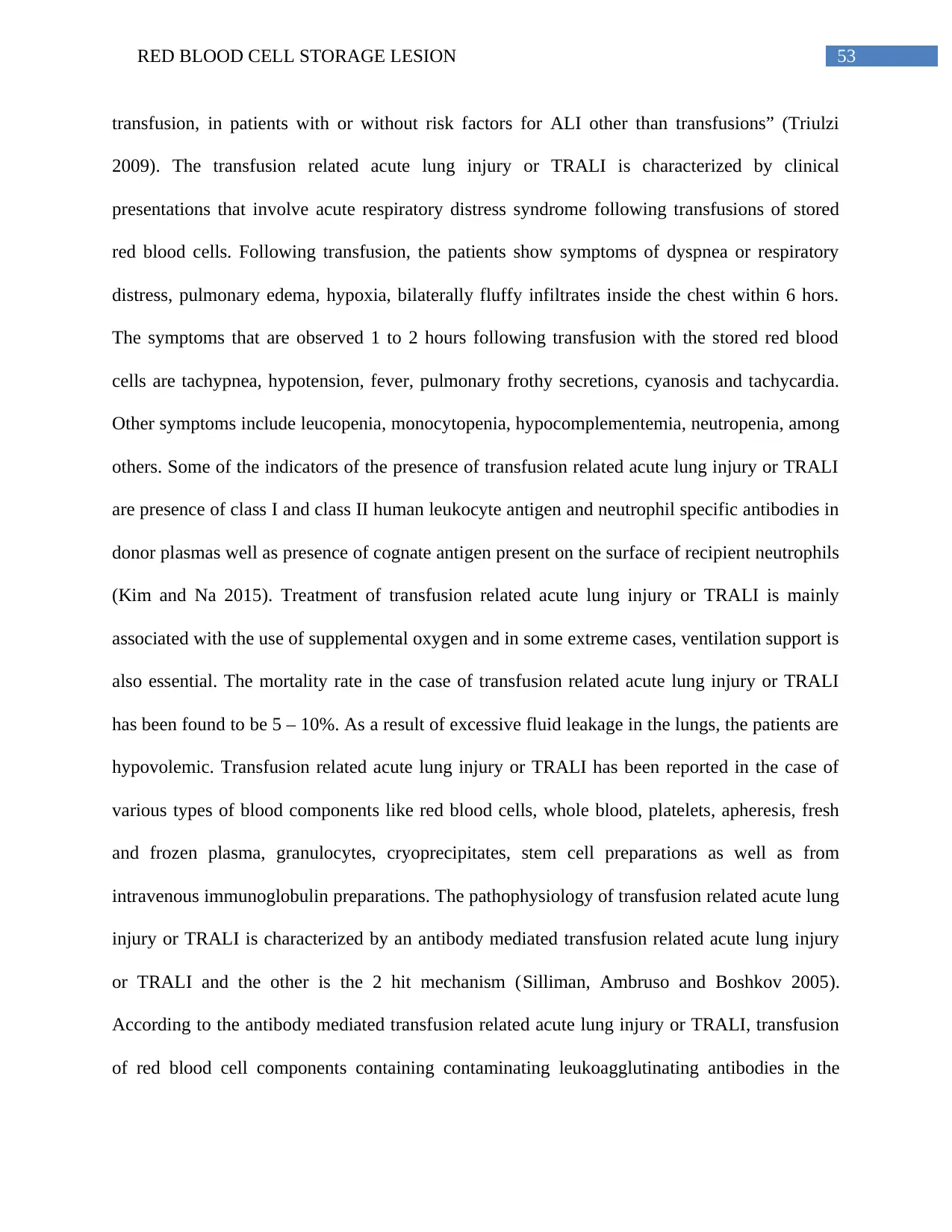
53RED BLOOD CELL STORAGE LESION
transfusion, in patients with or without risk factors for ALI other than transfusions” (Triulzi
2009). The transfusion related acute lung injury or TRALI is characterized by clinical
presentations that involve acute respiratory distress syndrome following transfusions of stored
red blood cells. Following transfusion, the patients show symptoms of dyspnea or respiratory
distress, pulmonary edema, hypoxia, bilaterally fluffy infiltrates inside the chest within 6 hors.
The symptoms that are observed 1 to 2 hours following transfusion with the stored red blood
cells are tachypnea, hypotension, fever, pulmonary frothy secretions, cyanosis and tachycardia.
Other symptoms include leucopenia, monocytopenia, hypocomplementemia, neutropenia, among
others. Some of the indicators of the presence of transfusion related acute lung injury or TRALI
are presence of class I and class II human leukocyte antigen and neutrophil specific antibodies in
donor plasmas well as presence of cognate antigen present on the surface of recipient neutrophils
(Kim and Na 2015). Treatment of transfusion related acute lung injury or TRALI is mainly
associated with the use of supplemental oxygen and in some extreme cases, ventilation support is
also essential. The mortality rate in the case of transfusion related acute lung injury or TRALI
has been found to be 5 – 10%. As a result of excessive fluid leakage in the lungs, the patients are
hypovolemic. Transfusion related acute lung injury or TRALI has been reported in the case of
various types of blood components like red blood cells, whole blood, platelets, apheresis, fresh
and frozen plasma, granulocytes, cryoprecipitates, stem cell preparations as well as from
intravenous immunoglobulin preparations. The pathophysiology of transfusion related acute lung
injury or TRALI is characterized by an antibody mediated transfusion related acute lung injury
or TRALI and the other is the 2 hit mechanism (Silliman, Ambruso and Boshkov 2005).
According to the antibody mediated transfusion related acute lung injury or TRALI, transfusion
of red blood cell components containing contaminating leukoagglutinating antibodies in the
transfusion, in patients with or without risk factors for ALI other than transfusions” (Triulzi
2009). The transfusion related acute lung injury or TRALI is characterized by clinical
presentations that involve acute respiratory distress syndrome following transfusions of stored
red blood cells. Following transfusion, the patients show symptoms of dyspnea or respiratory
distress, pulmonary edema, hypoxia, bilaterally fluffy infiltrates inside the chest within 6 hors.
The symptoms that are observed 1 to 2 hours following transfusion with the stored red blood
cells are tachypnea, hypotension, fever, pulmonary frothy secretions, cyanosis and tachycardia.
Other symptoms include leucopenia, monocytopenia, hypocomplementemia, neutropenia, among
others. Some of the indicators of the presence of transfusion related acute lung injury or TRALI
are presence of class I and class II human leukocyte antigen and neutrophil specific antibodies in
donor plasmas well as presence of cognate antigen present on the surface of recipient neutrophils
(Kim and Na 2015). Treatment of transfusion related acute lung injury or TRALI is mainly
associated with the use of supplemental oxygen and in some extreme cases, ventilation support is
also essential. The mortality rate in the case of transfusion related acute lung injury or TRALI
has been found to be 5 – 10%. As a result of excessive fluid leakage in the lungs, the patients are
hypovolemic. Transfusion related acute lung injury or TRALI has been reported in the case of
various types of blood components like red blood cells, whole blood, platelets, apheresis, fresh
and frozen plasma, granulocytes, cryoprecipitates, stem cell preparations as well as from
intravenous immunoglobulin preparations. The pathophysiology of transfusion related acute lung
injury or TRALI is characterized by an antibody mediated transfusion related acute lung injury
or TRALI and the other is the 2 hit mechanism (Silliman, Ambruso and Boshkov 2005).
According to the antibody mediated transfusion related acute lung injury or TRALI, transfusion
of red blood cell components containing contaminating leukoagglutinating antibodies in the
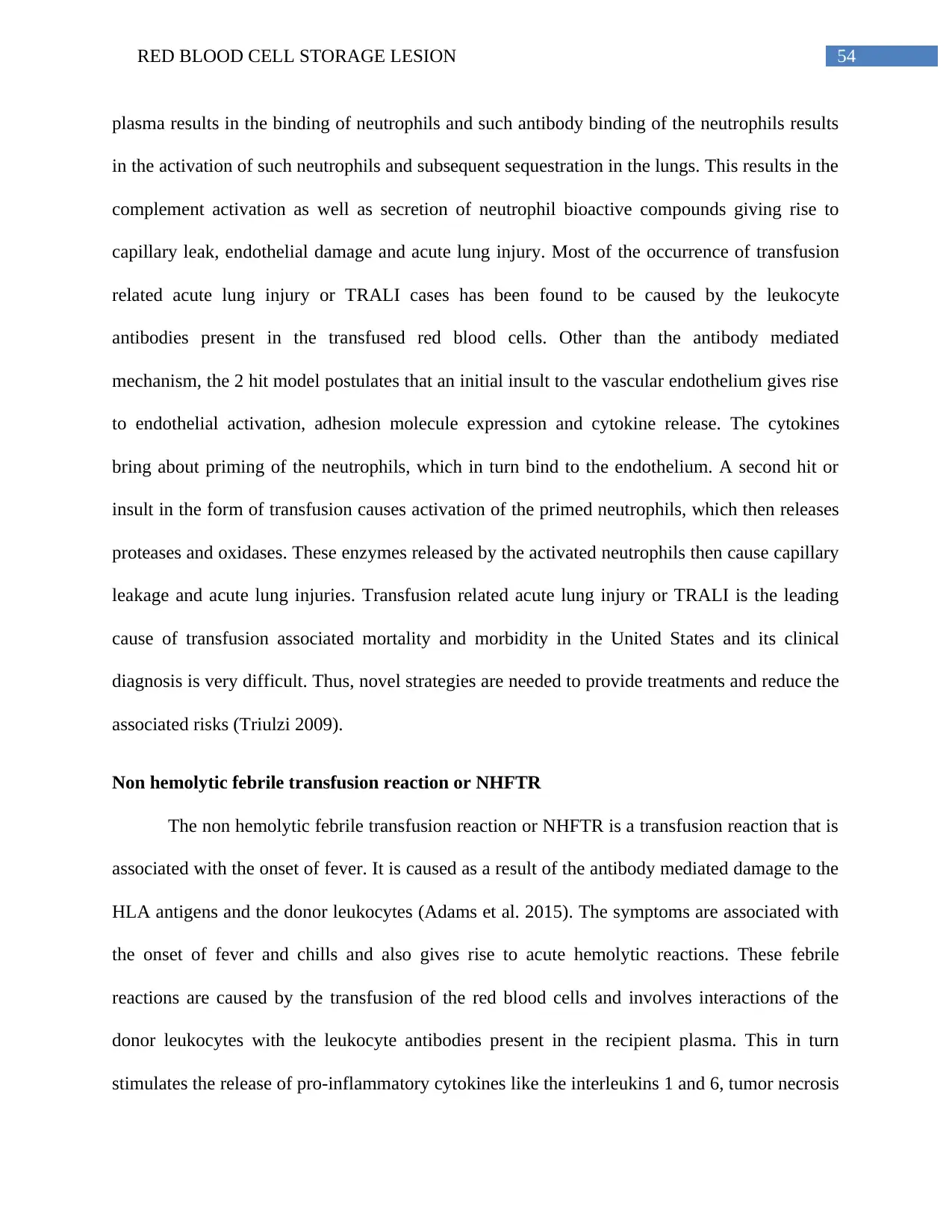
54RED BLOOD CELL STORAGE LESION
plasma results in the binding of neutrophils and such antibody binding of the neutrophils results
in the activation of such neutrophils and subsequent sequestration in the lungs. This results in the
complement activation as well as secretion of neutrophil bioactive compounds giving rise to
capillary leak, endothelial damage and acute lung injury. Most of the occurrence of transfusion
related acute lung injury or TRALI cases has been found to be caused by the leukocyte
antibodies present in the transfused red blood cells. Other than the antibody mediated
mechanism, the 2 hit model postulates that an initial insult to the vascular endothelium gives rise
to endothelial activation, adhesion molecule expression and cytokine release. The cytokines
bring about priming of the neutrophils, which in turn bind to the endothelium. A second hit or
insult in the form of transfusion causes activation of the primed neutrophils, which then releases
proteases and oxidases. These enzymes released by the activated neutrophils then cause capillary
leakage and acute lung injuries. Transfusion related acute lung injury or TRALI is the leading
cause of transfusion associated mortality and morbidity in the United States and its clinical
diagnosis is very difficult. Thus, novel strategies are needed to provide treatments and reduce the
associated risks (Triulzi 2009).
Non hemolytic febrile transfusion reaction or NHFTR
The non hemolytic febrile transfusion reaction or NHFTR is a transfusion reaction that is
associated with the onset of fever. It is caused as a result of the antibody mediated damage to the
HLA antigens and the donor leukocytes (Adams et al. 2015). The symptoms are associated with
the onset of fever and chills and also gives rise to acute hemolytic reactions. These febrile
reactions are caused by the transfusion of the red blood cells and involves interactions of the
donor leukocytes with the leukocyte antibodies present in the recipient plasma. This in turn
stimulates the release of pro-inflammatory cytokines like the interleukins 1 and 6, tumor necrosis
plasma results in the binding of neutrophils and such antibody binding of the neutrophils results
in the activation of such neutrophils and subsequent sequestration in the lungs. This results in the
complement activation as well as secretion of neutrophil bioactive compounds giving rise to
capillary leak, endothelial damage and acute lung injury. Most of the occurrence of transfusion
related acute lung injury or TRALI cases has been found to be caused by the leukocyte
antibodies present in the transfused red blood cells. Other than the antibody mediated
mechanism, the 2 hit model postulates that an initial insult to the vascular endothelium gives rise
to endothelial activation, adhesion molecule expression and cytokine release. The cytokines
bring about priming of the neutrophils, which in turn bind to the endothelium. A second hit or
insult in the form of transfusion causes activation of the primed neutrophils, which then releases
proteases and oxidases. These enzymes released by the activated neutrophils then cause capillary
leakage and acute lung injuries. Transfusion related acute lung injury or TRALI is the leading
cause of transfusion associated mortality and morbidity in the United States and its clinical
diagnosis is very difficult. Thus, novel strategies are needed to provide treatments and reduce the
associated risks (Triulzi 2009).
Non hemolytic febrile transfusion reaction or NHFTR
The non hemolytic febrile transfusion reaction or NHFTR is a transfusion reaction that is
associated with the onset of fever. It is caused as a result of the antibody mediated damage to the
HLA antigens and the donor leukocytes (Adams et al. 2015). The symptoms are associated with
the onset of fever and chills and also gives rise to acute hemolytic reactions. These febrile
reactions are caused by the transfusion of the red blood cells and involves interactions of the
donor leukocytes with the leukocyte antibodies present in the recipient plasma. This in turn
stimulates the release of pro-inflammatory cytokines like the interleukins 1 and 6, tumor necrosis
Paraphrase This Document
Need a fresh take? Get an instant paraphrase of this document with our AI Paraphraser
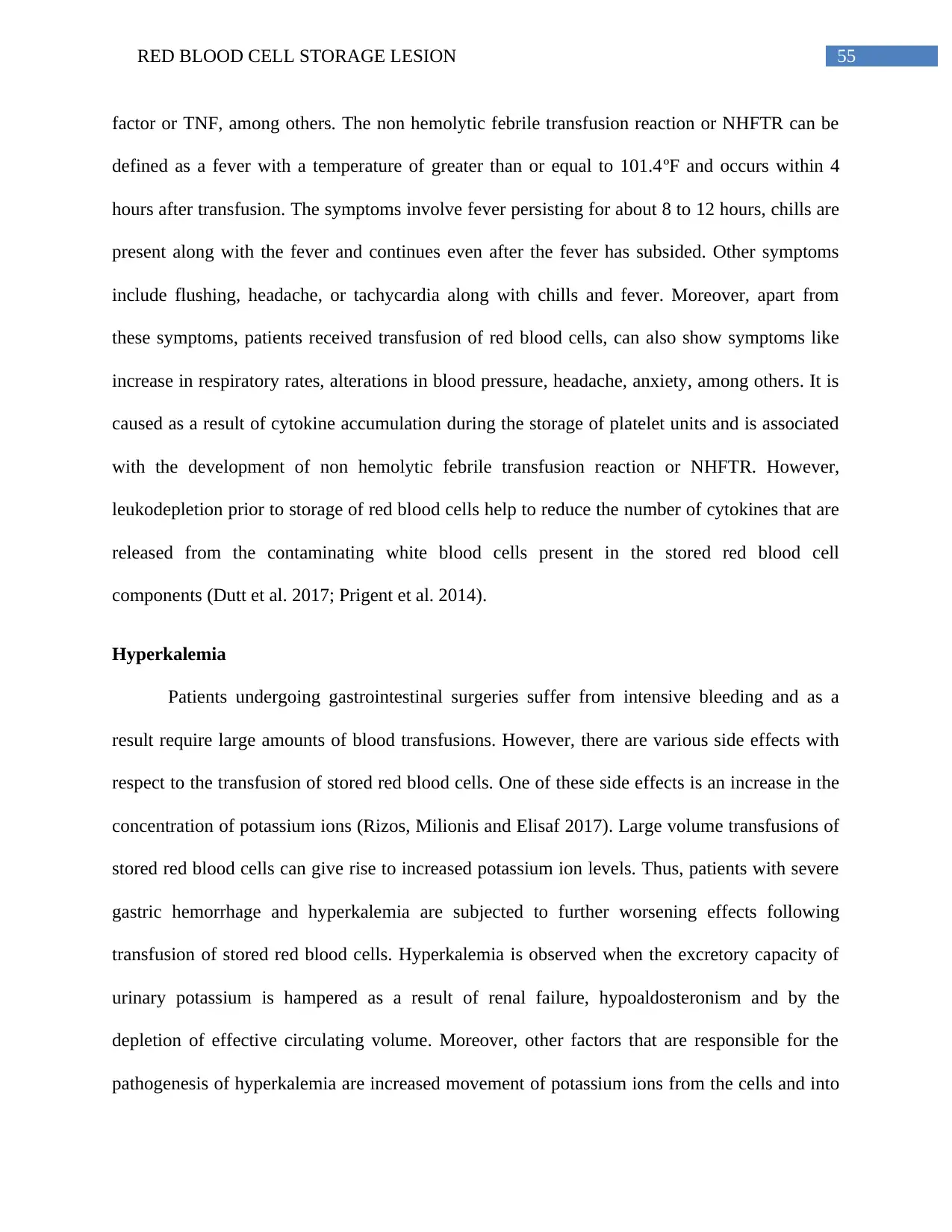
55RED BLOOD CELL STORAGE LESION
factor or TNF, among others. The non hemolytic febrile transfusion reaction or NHFTR can be
defined as a fever with a temperature of greater than or equal to 101.4ºF and occurs within 4
hours after transfusion. The symptoms involve fever persisting for about 8 to 12 hours, chills are
present along with the fever and continues even after the fever has subsided. Other symptoms
include flushing, headache, or tachycardia along with chills and fever. Moreover, apart from
these symptoms, patients received transfusion of red blood cells, can also show symptoms like
increase in respiratory rates, alterations in blood pressure, headache, anxiety, among others. It is
caused as a result of cytokine accumulation during the storage of platelet units and is associated
with the development of non hemolytic febrile transfusion reaction or NHFTR. However,
leukodepletion prior to storage of red blood cells help to reduce the number of cytokines that are
released from the contaminating white blood cells present in the stored red blood cell
components (Dutt et al. 2017; Prigent et al. 2014).
Hyperkalemia
Patients undergoing gastrointestinal surgeries suffer from intensive bleeding and as a
result require large amounts of blood transfusions. However, there are various side effects with
respect to the transfusion of stored red blood cells. One of these side effects is an increase in the
concentration of potassium ions (Rizos, Milionis and Elisaf 2017). Large volume transfusions of
stored red blood cells can give rise to increased potassium ion levels. Thus, patients with severe
gastric hemorrhage and hyperkalemia are subjected to further worsening effects following
transfusion of stored red blood cells. Hyperkalemia is observed when the excretory capacity of
urinary potassium is hampered as a result of renal failure, hypoaldosteronism and by the
depletion of effective circulating volume. Moreover, other factors that are responsible for the
pathogenesis of hyperkalemia are increased movement of potassium ions from the cells and into
factor or TNF, among others. The non hemolytic febrile transfusion reaction or NHFTR can be
defined as a fever with a temperature of greater than or equal to 101.4ºF and occurs within 4
hours after transfusion. The symptoms involve fever persisting for about 8 to 12 hours, chills are
present along with the fever and continues even after the fever has subsided. Other symptoms
include flushing, headache, or tachycardia along with chills and fever. Moreover, apart from
these symptoms, patients received transfusion of red blood cells, can also show symptoms like
increase in respiratory rates, alterations in blood pressure, headache, anxiety, among others. It is
caused as a result of cytokine accumulation during the storage of platelet units and is associated
with the development of non hemolytic febrile transfusion reaction or NHFTR. However,
leukodepletion prior to storage of red blood cells help to reduce the number of cytokines that are
released from the contaminating white blood cells present in the stored red blood cell
components (Dutt et al. 2017; Prigent et al. 2014).
Hyperkalemia
Patients undergoing gastrointestinal surgeries suffer from intensive bleeding and as a
result require large amounts of blood transfusions. However, there are various side effects with
respect to the transfusion of stored red blood cells. One of these side effects is an increase in the
concentration of potassium ions (Rizos, Milionis and Elisaf 2017). Large volume transfusions of
stored red blood cells can give rise to increased potassium ion levels. Thus, patients with severe
gastric hemorrhage and hyperkalemia are subjected to further worsening effects following
transfusion of stored red blood cells. Hyperkalemia is observed when the excretory capacity of
urinary potassium is hampered as a result of renal failure, hypoaldosteronism and by the
depletion of effective circulating volume. Moreover, other factors that are responsible for the
pathogenesis of hyperkalemia are increased movement of potassium ions from the cells and into
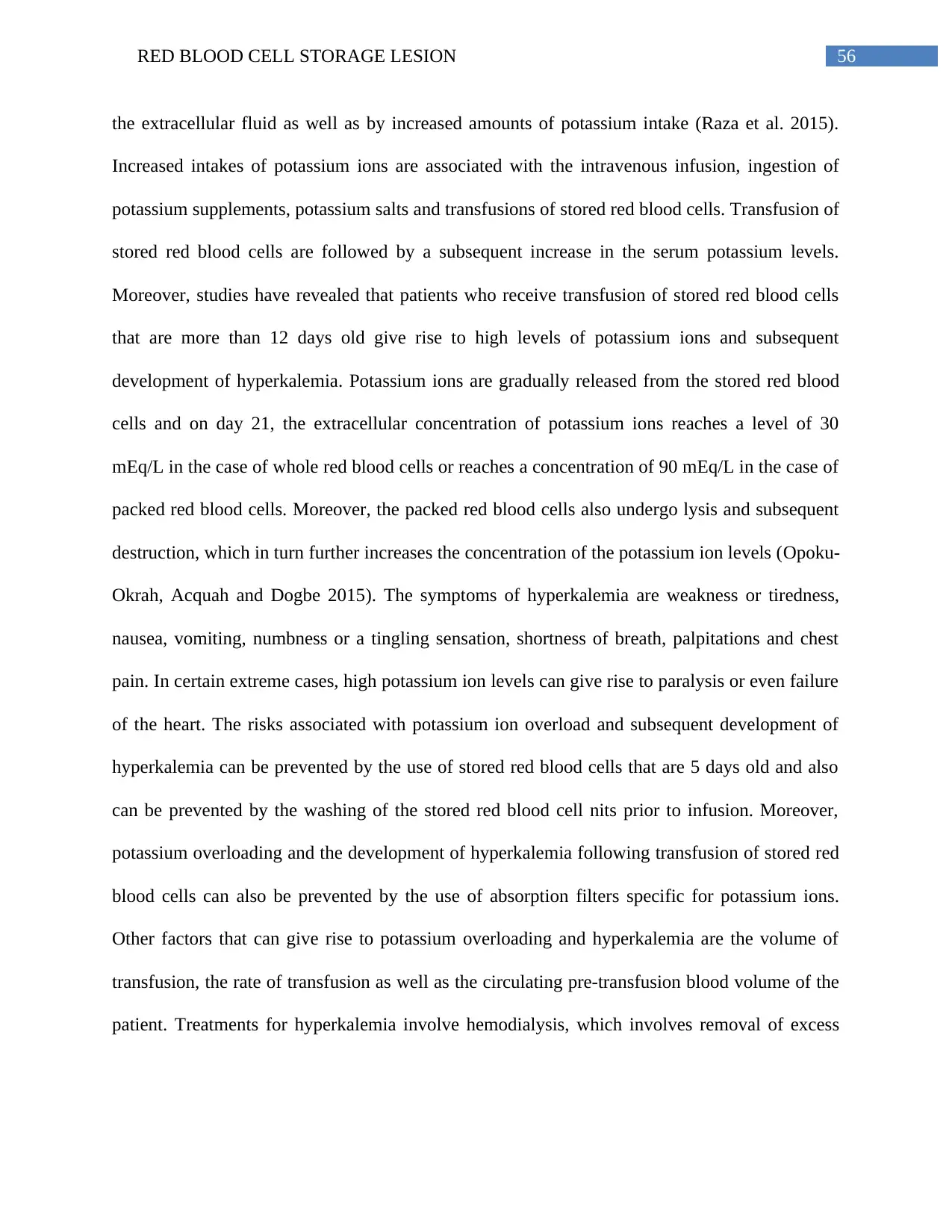
56RED BLOOD CELL STORAGE LESION
the extracellular fluid as well as by increased amounts of potassium intake (Raza et al. 2015).
Increased intakes of potassium ions are associated with the intravenous infusion, ingestion of
potassium supplements, potassium salts and transfusions of stored red blood cells. Transfusion of
stored red blood cells are followed by a subsequent increase in the serum potassium levels.
Moreover, studies have revealed that patients who receive transfusion of stored red blood cells
that are more than 12 days old give rise to high levels of potassium ions and subsequent
development of hyperkalemia. Potassium ions are gradually released from the stored red blood
cells and on day 21, the extracellular concentration of potassium ions reaches a level of 30
mEq/L in the case of whole red blood cells or reaches a concentration of 90 mEq/L in the case of
packed red blood cells. Moreover, the packed red blood cells also undergo lysis and subsequent
destruction, which in turn further increases the concentration of the potassium ion levels (Opoku-
Okrah, Acquah and Dogbe 2015). The symptoms of hyperkalemia are weakness or tiredness,
nausea, vomiting, numbness or a tingling sensation, shortness of breath, palpitations and chest
pain. In certain extreme cases, high potassium ion levels can give rise to paralysis or even failure
of the heart. The risks associated with potassium ion overload and subsequent development of
hyperkalemia can be prevented by the use of stored red blood cells that are 5 days old and also
can be prevented by the washing of the stored red blood cell nits prior to infusion. Moreover,
potassium overloading and the development of hyperkalemia following transfusion of stored red
blood cells can also be prevented by the use of absorption filters specific for potassium ions.
Other factors that can give rise to potassium overloading and hyperkalemia are the volume of
transfusion, the rate of transfusion as well as the circulating pre-transfusion blood volume of the
patient. Treatments for hyperkalemia involve hemodialysis, which involves removal of excess
the extracellular fluid as well as by increased amounts of potassium intake (Raza et al. 2015).
Increased intakes of potassium ions are associated with the intravenous infusion, ingestion of
potassium supplements, potassium salts and transfusions of stored red blood cells. Transfusion of
stored red blood cells are followed by a subsequent increase in the serum potassium levels.
Moreover, studies have revealed that patients who receive transfusion of stored red blood cells
that are more than 12 days old give rise to high levels of potassium ions and subsequent
development of hyperkalemia. Potassium ions are gradually released from the stored red blood
cells and on day 21, the extracellular concentration of potassium ions reaches a level of 30
mEq/L in the case of whole red blood cells or reaches a concentration of 90 mEq/L in the case of
packed red blood cells. Moreover, the packed red blood cells also undergo lysis and subsequent
destruction, which in turn further increases the concentration of the potassium ion levels (Opoku-
Okrah, Acquah and Dogbe 2015). The symptoms of hyperkalemia are weakness or tiredness,
nausea, vomiting, numbness or a tingling sensation, shortness of breath, palpitations and chest
pain. In certain extreme cases, high potassium ion levels can give rise to paralysis or even failure
of the heart. The risks associated with potassium ion overload and subsequent development of
hyperkalemia can be prevented by the use of stored red blood cells that are 5 days old and also
can be prevented by the washing of the stored red blood cell nits prior to infusion. Moreover,
potassium overloading and the development of hyperkalemia following transfusion of stored red
blood cells can also be prevented by the use of absorption filters specific for potassium ions.
Other factors that can give rise to potassium overloading and hyperkalemia are the volume of
transfusion, the rate of transfusion as well as the circulating pre-transfusion blood volume of the
patient. Treatments for hyperkalemia involve hemodialysis, which involves removal of excess
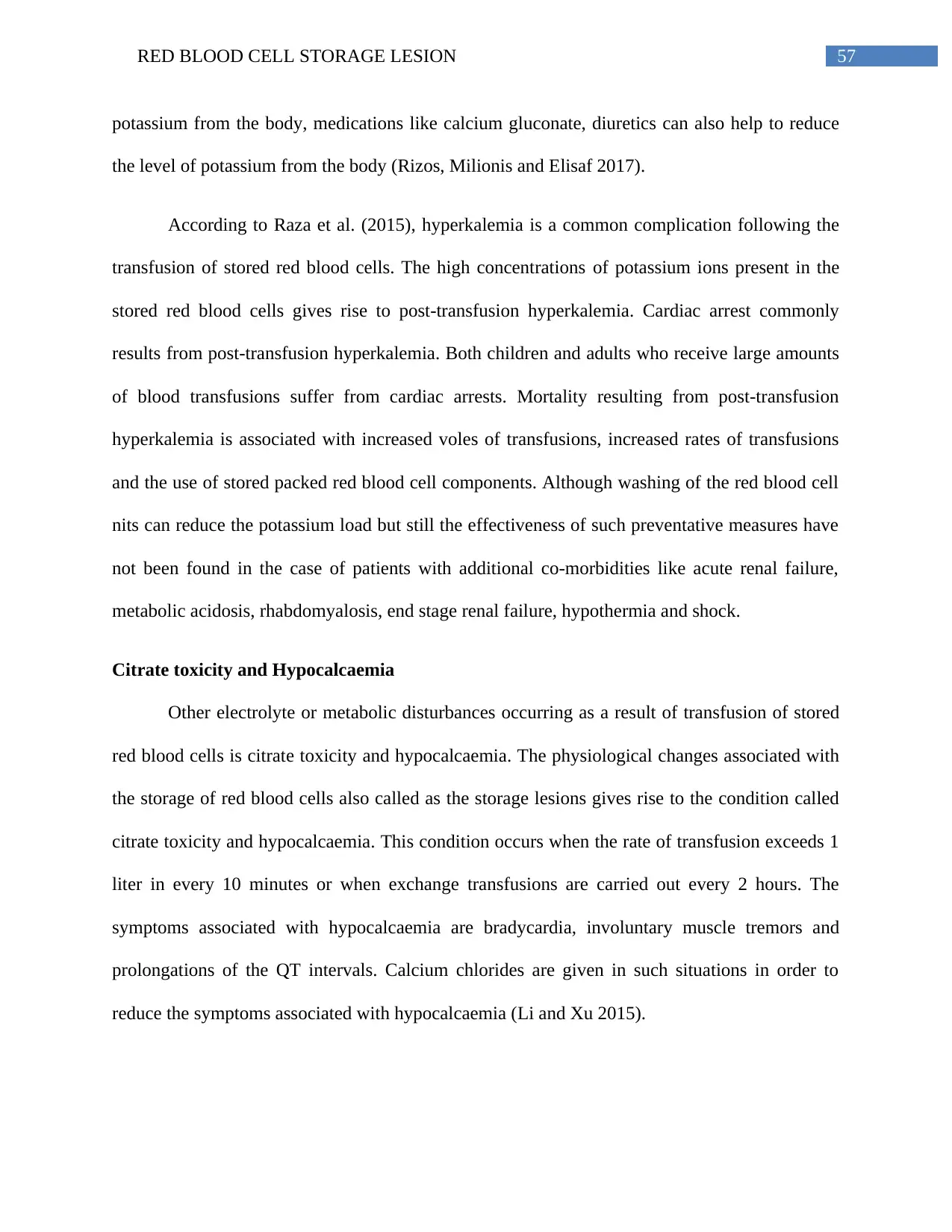
57RED BLOOD CELL STORAGE LESION
potassium from the body, medications like calcium gluconate, diuretics can also help to reduce
the level of potassium from the body (Rizos, Milionis and Elisaf 2017).
According to Raza et al. (2015), hyperkalemia is a common complication following the
transfusion of stored red blood cells. The high concentrations of potassium ions present in the
stored red blood cells gives rise to post-transfusion hyperkalemia. Cardiac arrest commonly
results from post-transfusion hyperkalemia. Both children and adults who receive large amounts
of blood transfusions suffer from cardiac arrests. Mortality resulting from post-transfusion
hyperkalemia is associated with increased voles of transfusions, increased rates of transfusions
and the use of stored packed red blood cell components. Although washing of the red blood cell
nits can reduce the potassium load but still the effectiveness of such preventative measures have
not been found in the case of patients with additional co-morbidities like acute renal failure,
metabolic acidosis, rhabdomyalosis, end stage renal failure, hypothermia and shock.
Citrate toxicity and Hypocalcaemia
Other electrolyte or metabolic disturbances occurring as a result of transfusion of stored
red blood cells is citrate toxicity and hypocalcaemia. The physiological changes associated with
the storage of red blood cells also called as the storage lesions gives rise to the condition called
citrate toxicity and hypocalcaemia. This condition occurs when the rate of transfusion exceeds 1
liter in every 10 minutes or when exchange transfusions are carried out every 2 hours. The
symptoms associated with hypocalcaemia are bradycardia, involuntary muscle tremors and
prolongations of the QT intervals. Calcium chlorides are given in such situations in order to
reduce the symptoms associated with hypocalcaemia (Li and Xu 2015).
potassium from the body, medications like calcium gluconate, diuretics can also help to reduce
the level of potassium from the body (Rizos, Milionis and Elisaf 2017).
According to Raza et al. (2015), hyperkalemia is a common complication following the
transfusion of stored red blood cells. The high concentrations of potassium ions present in the
stored red blood cells gives rise to post-transfusion hyperkalemia. Cardiac arrest commonly
results from post-transfusion hyperkalemia. Both children and adults who receive large amounts
of blood transfusions suffer from cardiac arrests. Mortality resulting from post-transfusion
hyperkalemia is associated with increased voles of transfusions, increased rates of transfusions
and the use of stored packed red blood cell components. Although washing of the red blood cell
nits can reduce the potassium load but still the effectiveness of such preventative measures have
not been found in the case of patients with additional co-morbidities like acute renal failure,
metabolic acidosis, rhabdomyalosis, end stage renal failure, hypothermia and shock.
Citrate toxicity and Hypocalcaemia
Other electrolyte or metabolic disturbances occurring as a result of transfusion of stored
red blood cells is citrate toxicity and hypocalcaemia. The physiological changes associated with
the storage of red blood cells also called as the storage lesions gives rise to the condition called
citrate toxicity and hypocalcaemia. This condition occurs when the rate of transfusion exceeds 1
liter in every 10 minutes or when exchange transfusions are carried out every 2 hours. The
symptoms associated with hypocalcaemia are bradycardia, involuntary muscle tremors and
prolongations of the QT intervals. Calcium chlorides are given in such situations in order to
reduce the symptoms associated with hypocalcaemia (Li and Xu 2015).
Secure Best Marks with AI Grader
Need help grading? Try our AI Grader for instant feedback on your assignments.
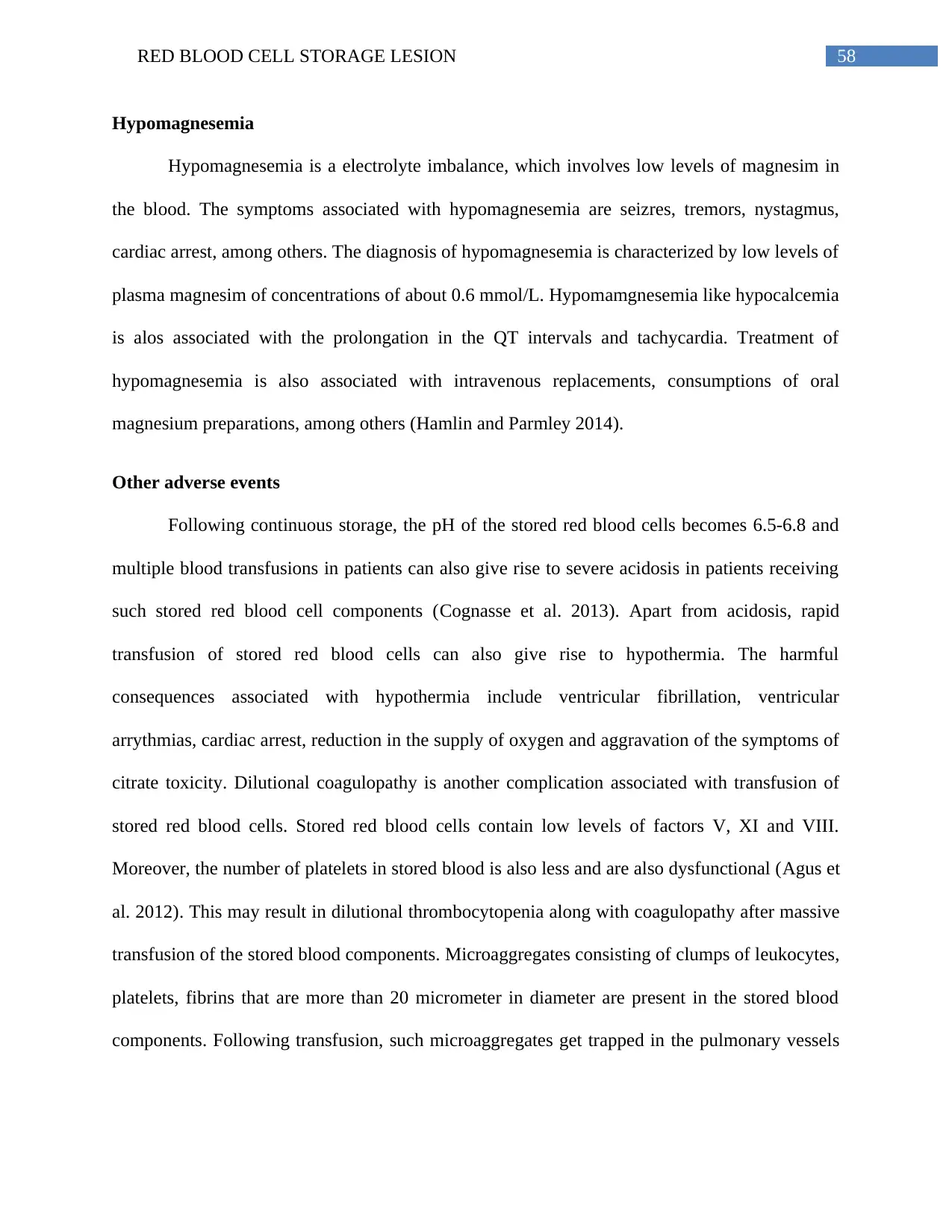
58RED BLOOD CELL STORAGE LESION
Hypomagnesemia
Hypomagnesemia is a electrolyte imbalance, which involves low levels of magnesim in
the blood. The symptoms associated with hypomagnesemia are seizres, tremors, nystagmus,
cardiac arrest, among others. The diagnosis of hypomagnesemia is characterized by low levels of
plasma magnesim of concentrations of about 0.6 mmol/L. Hypomamgnesemia like hypocalcemia
is alos associated with the prolongation in the QT intervals and tachycardia. Treatment of
hypomagnesemia is also associated with intravenous replacements, consumptions of oral
magnesium preparations, among others (Hamlin and Parmley 2014).
Other adverse events
Following continuous storage, the pH of the stored red blood cells becomes 6.5-6.8 and
multiple blood transfusions in patients can also give rise to severe acidosis in patients receiving
such stored red blood cell components (Cognasse et al. 2013). Apart from acidosis, rapid
transfusion of stored red blood cells can also give rise to hypothermia. The harmful
consequences associated with hypothermia include ventricular fibrillation, ventricular
arrythmias, cardiac arrest, reduction in the supply of oxygen and aggravation of the symptoms of
citrate toxicity. Dilutional coagulopathy is another complication associated with transfusion of
stored red blood cells. Stored red blood cells contain low levels of factors V, XI and VIII.
Moreover, the number of platelets in stored blood is also less and are also dysfunctional (Agus et
al. 2012). This may result in dilutional thrombocytopenia along with coagulopathy after massive
transfusion of the stored blood components. Microaggregates consisting of clumps of leukocytes,
platelets, fibrins that are more than 20 micrometer in diameter are present in the stored blood
components. Following transfusion, such microaggregates get trapped in the pulmonary vessels
Hypomagnesemia
Hypomagnesemia is a electrolyte imbalance, which involves low levels of magnesim in
the blood. The symptoms associated with hypomagnesemia are seizres, tremors, nystagmus,
cardiac arrest, among others. The diagnosis of hypomagnesemia is characterized by low levels of
plasma magnesim of concentrations of about 0.6 mmol/L. Hypomamgnesemia like hypocalcemia
is alos associated with the prolongation in the QT intervals and tachycardia. Treatment of
hypomagnesemia is also associated with intravenous replacements, consumptions of oral
magnesium preparations, among others (Hamlin and Parmley 2014).
Other adverse events
Following continuous storage, the pH of the stored red blood cells becomes 6.5-6.8 and
multiple blood transfusions in patients can also give rise to severe acidosis in patients receiving
such stored red blood cell components (Cognasse et al. 2013). Apart from acidosis, rapid
transfusion of stored red blood cells can also give rise to hypothermia. The harmful
consequences associated with hypothermia include ventricular fibrillation, ventricular
arrythmias, cardiac arrest, reduction in the supply of oxygen and aggravation of the symptoms of
citrate toxicity. Dilutional coagulopathy is another complication associated with transfusion of
stored red blood cells. Stored red blood cells contain low levels of factors V, XI and VIII.
Moreover, the number of platelets in stored blood is also less and are also dysfunctional (Agus et
al. 2012). This may result in dilutional thrombocytopenia along with coagulopathy after massive
transfusion of the stored blood components. Microaggregates consisting of clumps of leukocytes,
platelets, fibrins that are more than 20 micrometer in diameter are present in the stored blood
components. Following transfusion, such microaggregates get trapped in the pulmonary vessels
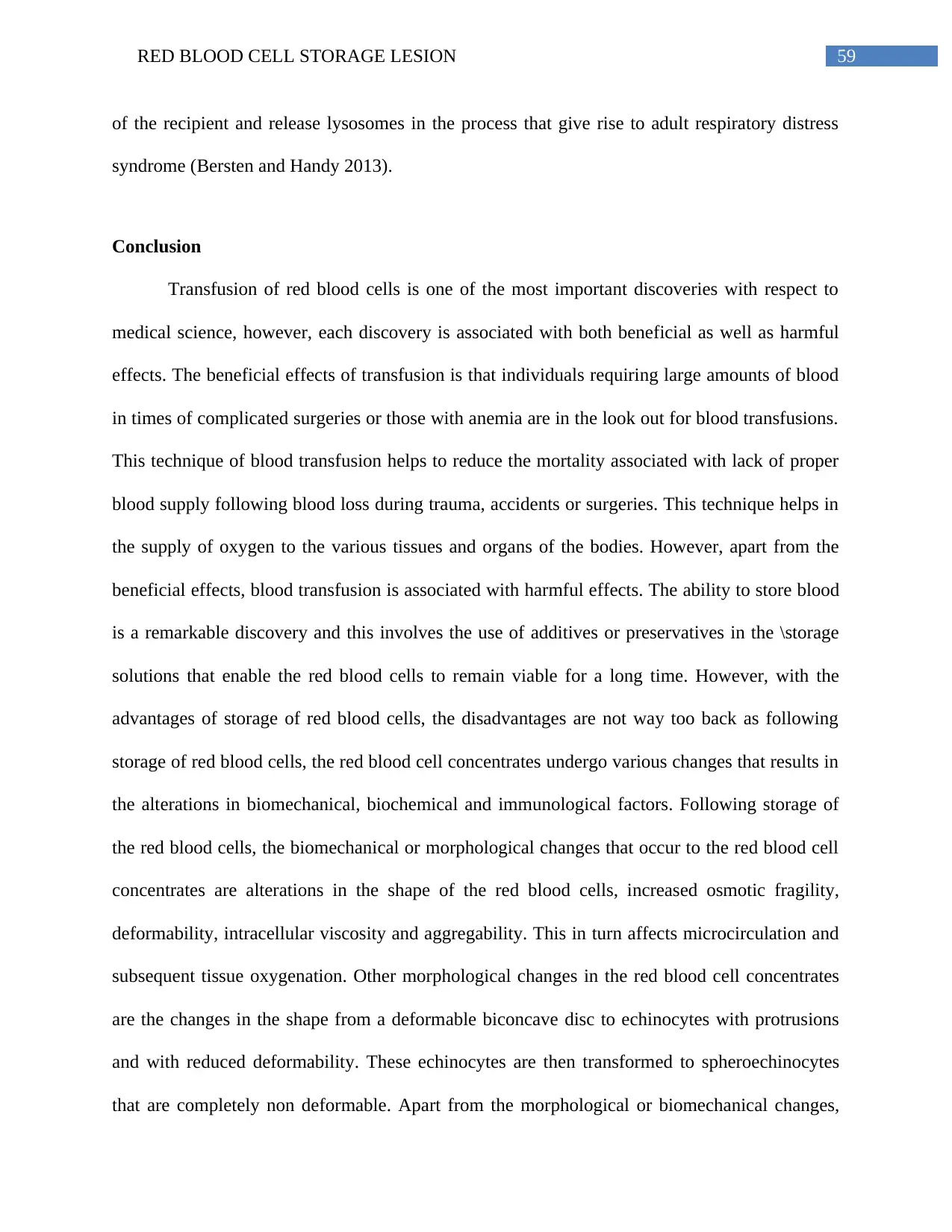
59RED BLOOD CELL STORAGE LESION
of the recipient and release lysosomes in the process that give rise to adult respiratory distress
syndrome (Bersten and Handy 2013).
Conclusion
Transfusion of red blood cells is one of the most important discoveries with respect to
medical science, however, each discovery is associated with both beneficial as well as harmful
effects. The beneficial effects of transfusion is that individuals requiring large amounts of blood
in times of complicated surgeries or those with anemia are in the look out for blood transfusions.
This technique of blood transfusion helps to reduce the mortality associated with lack of proper
blood supply following blood loss during trauma, accidents or surgeries. This technique helps in
the supply of oxygen to the various tissues and organs of the bodies. However, apart from the
beneficial effects, blood transfusion is associated with harmful effects. The ability to store blood
is a remarkable discovery and this involves the use of additives or preservatives in the \storage
solutions that enable the red blood cells to remain viable for a long time. However, with the
advantages of storage of red blood cells, the disadvantages are not way too back as following
storage of red blood cells, the red blood cell concentrates undergo various changes that results in
the alterations in biomechanical, biochemical and immunological factors. Following storage of
the red blood cells, the biomechanical or morphological changes that occur to the red blood cell
concentrates are alterations in the shape of the red blood cells, increased osmotic fragility,
deformability, intracellular viscosity and aggregability. This in turn affects microcirculation and
subsequent tissue oxygenation. Other morphological changes in the red blood cell concentrates
are the changes in the shape from a deformable biconcave disc to echinocytes with protrusions
and with reduced deformability. These echinocytes are then transformed to spheroechinocytes
that are completely non deformable. Apart from the morphological or biomechanical changes,
of the recipient and release lysosomes in the process that give rise to adult respiratory distress
syndrome (Bersten and Handy 2013).
Conclusion
Transfusion of red blood cells is one of the most important discoveries with respect to
medical science, however, each discovery is associated with both beneficial as well as harmful
effects. The beneficial effects of transfusion is that individuals requiring large amounts of blood
in times of complicated surgeries or those with anemia are in the look out for blood transfusions.
This technique of blood transfusion helps to reduce the mortality associated with lack of proper
blood supply following blood loss during trauma, accidents or surgeries. This technique helps in
the supply of oxygen to the various tissues and organs of the bodies. However, apart from the
beneficial effects, blood transfusion is associated with harmful effects. The ability to store blood
is a remarkable discovery and this involves the use of additives or preservatives in the \storage
solutions that enable the red blood cells to remain viable for a long time. However, with the
advantages of storage of red blood cells, the disadvantages are not way too back as following
storage of red blood cells, the red blood cell concentrates undergo various changes that results in
the alterations in biomechanical, biochemical and immunological factors. Following storage of
the red blood cells, the biomechanical or morphological changes that occur to the red blood cell
concentrates are alterations in the shape of the red blood cells, increased osmotic fragility,
deformability, intracellular viscosity and aggregability. This in turn affects microcirculation and
subsequent tissue oxygenation. Other morphological changes in the red blood cell concentrates
are the changes in the shape from a deformable biconcave disc to echinocytes with protrusions
and with reduced deformability. These echinocytes are then transformed to spheroechinocytes
that are completely non deformable. Apart from the morphological or biomechanical changes,
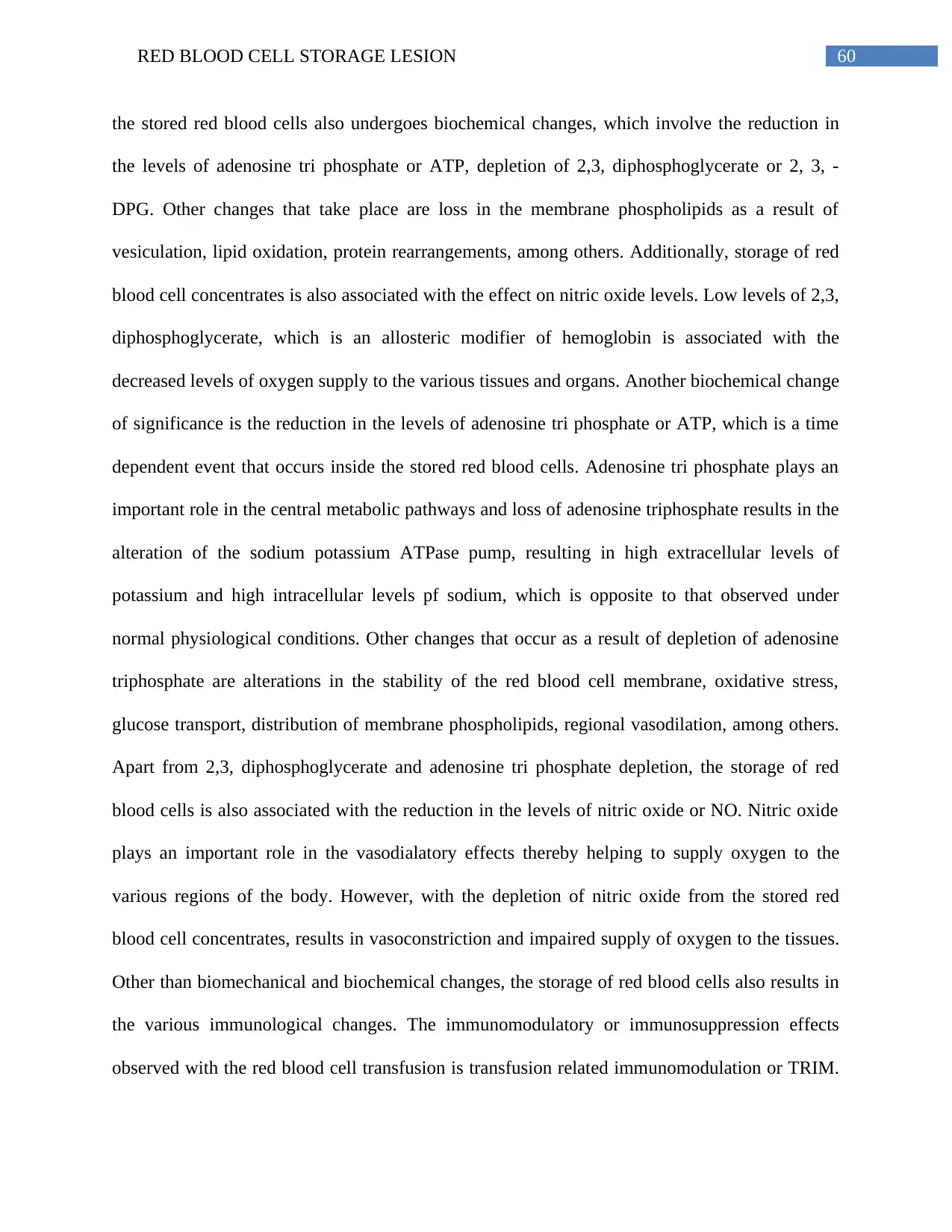
60RED BLOOD CELL STORAGE LESION
the stored red blood cells also undergoes biochemical changes, which involve the reduction in
the levels of adenosine tri phosphate or ATP, depletion of 2,3, diphosphoglycerate or 2, 3, -
DPG. Other changes that take place are loss in the membrane phospholipids as a result of
vesiculation, lipid oxidation, protein rearrangements, among others. Additionally, storage of red
blood cell concentrates is also associated with the effect on nitric oxide levels. Low levels of 2,3,
diphosphoglycerate, which is an allosteric modifier of hemoglobin is associated with the
decreased levels of oxygen supply to the various tissues and organs. Another biochemical change
of significance is the reduction in the levels of adenosine tri phosphate or ATP, which is a time
dependent event that occurs inside the stored red blood cells. Adenosine tri phosphate plays an
important role in the central metabolic pathways and loss of adenosine triphosphate results in the
alteration of the sodium potassium ATPase pump, resulting in high extracellular levels of
potassium and high intracellular levels pf sodium, which is opposite to that observed under
normal physiological conditions. Other changes that occur as a result of depletion of adenosine
triphosphate are alterations in the stability of the red blood cell membrane, oxidative stress,
glucose transport, distribution of membrane phospholipids, regional vasodilation, among others.
Apart from 2,3, diphosphoglycerate and adenosine tri phosphate depletion, the storage of red
blood cells is also associated with the reduction in the levels of nitric oxide or NO. Nitric oxide
plays an important role in the vasodialatory effects thereby helping to supply oxygen to the
various regions of the body. However, with the depletion of nitric oxide from the stored red
blood cell concentrates, results in vasoconstriction and impaired supply of oxygen to the tissues.
Other than biomechanical and biochemical changes, the storage of red blood cells also results in
the various immunological changes. The immunomodulatory or immunosuppression effects
observed with the red blood cell transfusion is transfusion related immunomodulation or TRIM.
the stored red blood cells also undergoes biochemical changes, which involve the reduction in
the levels of adenosine tri phosphate or ATP, depletion of 2,3, diphosphoglycerate or 2, 3, -
DPG. Other changes that take place are loss in the membrane phospholipids as a result of
vesiculation, lipid oxidation, protein rearrangements, among others. Additionally, storage of red
blood cell concentrates is also associated with the effect on nitric oxide levels. Low levels of 2,3,
diphosphoglycerate, which is an allosteric modifier of hemoglobin is associated with the
decreased levels of oxygen supply to the various tissues and organs. Another biochemical change
of significance is the reduction in the levels of adenosine tri phosphate or ATP, which is a time
dependent event that occurs inside the stored red blood cells. Adenosine tri phosphate plays an
important role in the central metabolic pathways and loss of adenosine triphosphate results in the
alteration of the sodium potassium ATPase pump, resulting in high extracellular levels of
potassium and high intracellular levels pf sodium, which is opposite to that observed under
normal physiological conditions. Other changes that occur as a result of depletion of adenosine
triphosphate are alterations in the stability of the red blood cell membrane, oxidative stress,
glucose transport, distribution of membrane phospholipids, regional vasodilation, among others.
Apart from 2,3, diphosphoglycerate and adenosine tri phosphate depletion, the storage of red
blood cells is also associated with the reduction in the levels of nitric oxide or NO. Nitric oxide
plays an important role in the vasodialatory effects thereby helping to supply oxygen to the
various regions of the body. However, with the depletion of nitric oxide from the stored red
blood cell concentrates, results in vasoconstriction and impaired supply of oxygen to the tissues.
Other than biomechanical and biochemical changes, the storage of red blood cells also results in
the various immunological changes. The immunomodulatory or immunosuppression effects
observed with the red blood cell transfusion is transfusion related immunomodulation or TRIM.
Paraphrase This Document
Need a fresh take? Get an instant paraphrase of this document with our AI Paraphraser
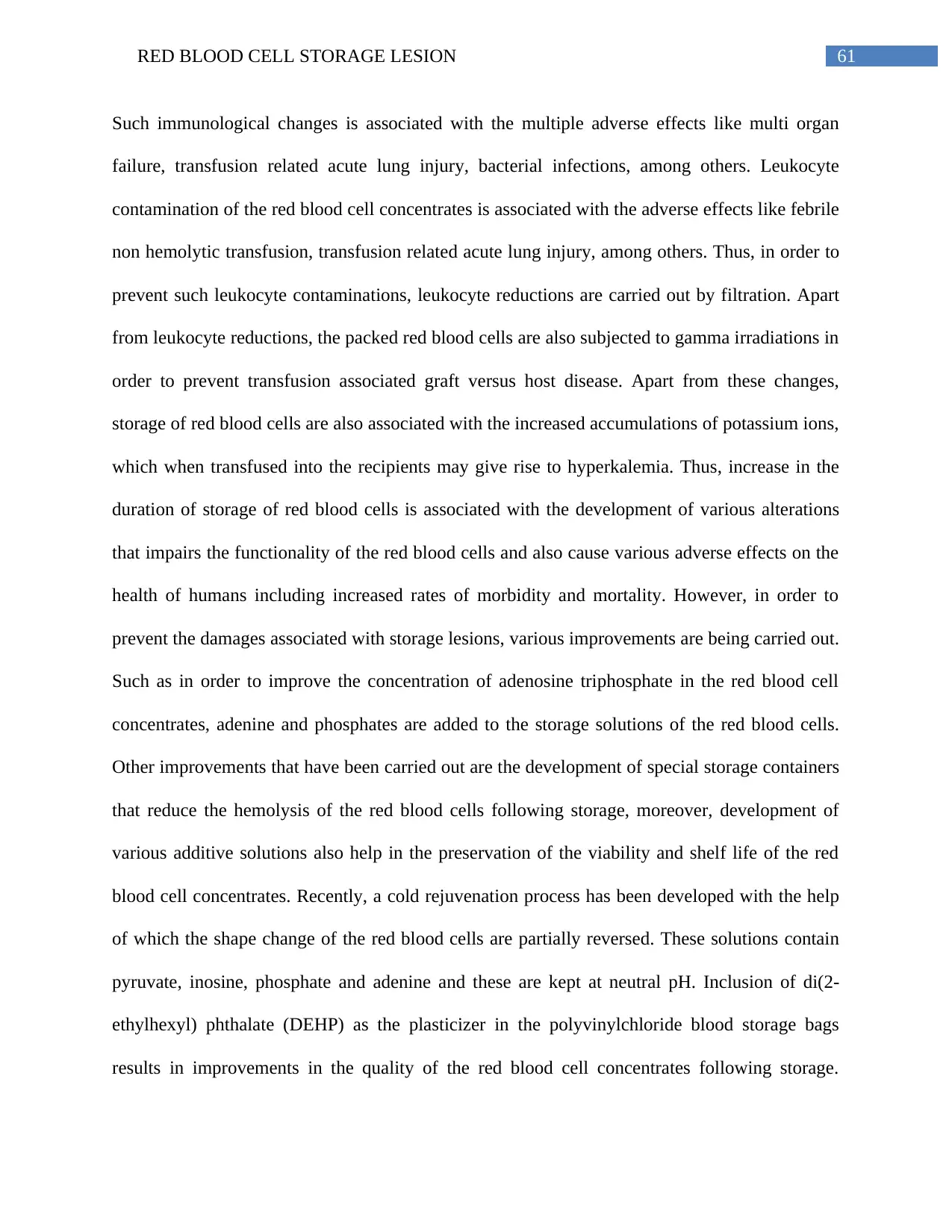
61RED BLOOD CELL STORAGE LESION
Such immunological changes is associated with the multiple adverse effects like multi organ
failure, transfusion related acute lung injury, bacterial infections, among others. Leukocyte
contamination of the red blood cell concentrates is associated with the adverse effects like febrile
non hemolytic transfusion, transfusion related acute lung injury, among others. Thus, in order to
prevent such leukocyte contaminations, leukocyte reductions are carried out by filtration. Apart
from leukocyte reductions, the packed red blood cells are also subjected to gamma irradiations in
order to prevent transfusion associated graft versus host disease. Apart from these changes,
storage of red blood cells are also associated with the increased accumulations of potassium ions,
which when transfused into the recipients may give rise to hyperkalemia. Thus, increase in the
duration of storage of red blood cells is associated with the development of various alterations
that impairs the functionality of the red blood cells and also cause various adverse effects on the
health of humans including increased rates of morbidity and mortality. However, in order to
prevent the damages associated with storage lesions, various improvements are being carried out.
Such as in order to improve the concentration of adenosine triphosphate in the red blood cell
concentrates, adenine and phosphates are added to the storage solutions of the red blood cells.
Other improvements that have been carried out are the development of special storage containers
that reduce the hemolysis of the red blood cells following storage, moreover, development of
various additive solutions also help in the preservation of the viability and shelf life of the red
blood cell concentrates. Recently, a cold rejuvenation process has been developed with the help
of which the shape change of the red blood cells are partially reversed. These solutions contain
pyruvate, inosine, phosphate and adenine and these are kept at neutral pH. Inclusion of di(2-
ethylhexyl) phthalate (DEHP) as the plasticizer in the polyvinylchloride blood storage bags
results in improvements in the quality of the red blood cell concentrates following storage.
Such immunological changes is associated with the multiple adverse effects like multi organ
failure, transfusion related acute lung injury, bacterial infections, among others. Leukocyte
contamination of the red blood cell concentrates is associated with the adverse effects like febrile
non hemolytic transfusion, transfusion related acute lung injury, among others. Thus, in order to
prevent such leukocyte contaminations, leukocyte reductions are carried out by filtration. Apart
from leukocyte reductions, the packed red blood cells are also subjected to gamma irradiations in
order to prevent transfusion associated graft versus host disease. Apart from these changes,
storage of red blood cells are also associated with the increased accumulations of potassium ions,
which when transfused into the recipients may give rise to hyperkalemia. Thus, increase in the
duration of storage of red blood cells is associated with the development of various alterations
that impairs the functionality of the red blood cells and also cause various adverse effects on the
health of humans including increased rates of morbidity and mortality. However, in order to
prevent the damages associated with storage lesions, various improvements are being carried out.
Such as in order to improve the concentration of adenosine triphosphate in the red blood cell
concentrates, adenine and phosphates are added to the storage solutions of the red blood cells.
Other improvements that have been carried out are the development of special storage containers
that reduce the hemolysis of the red blood cells following storage, moreover, development of
various additive solutions also help in the preservation of the viability and shelf life of the red
blood cell concentrates. Recently, a cold rejuvenation process has been developed with the help
of which the shape change of the red blood cells are partially reversed. These solutions contain
pyruvate, inosine, phosphate and adenine and these are kept at neutral pH. Inclusion of di(2-
ethylhexyl) phthalate (DEHP) as the plasticizer in the polyvinylchloride blood storage bags
results in improvements in the quality of the red blood cell concentrates following storage.
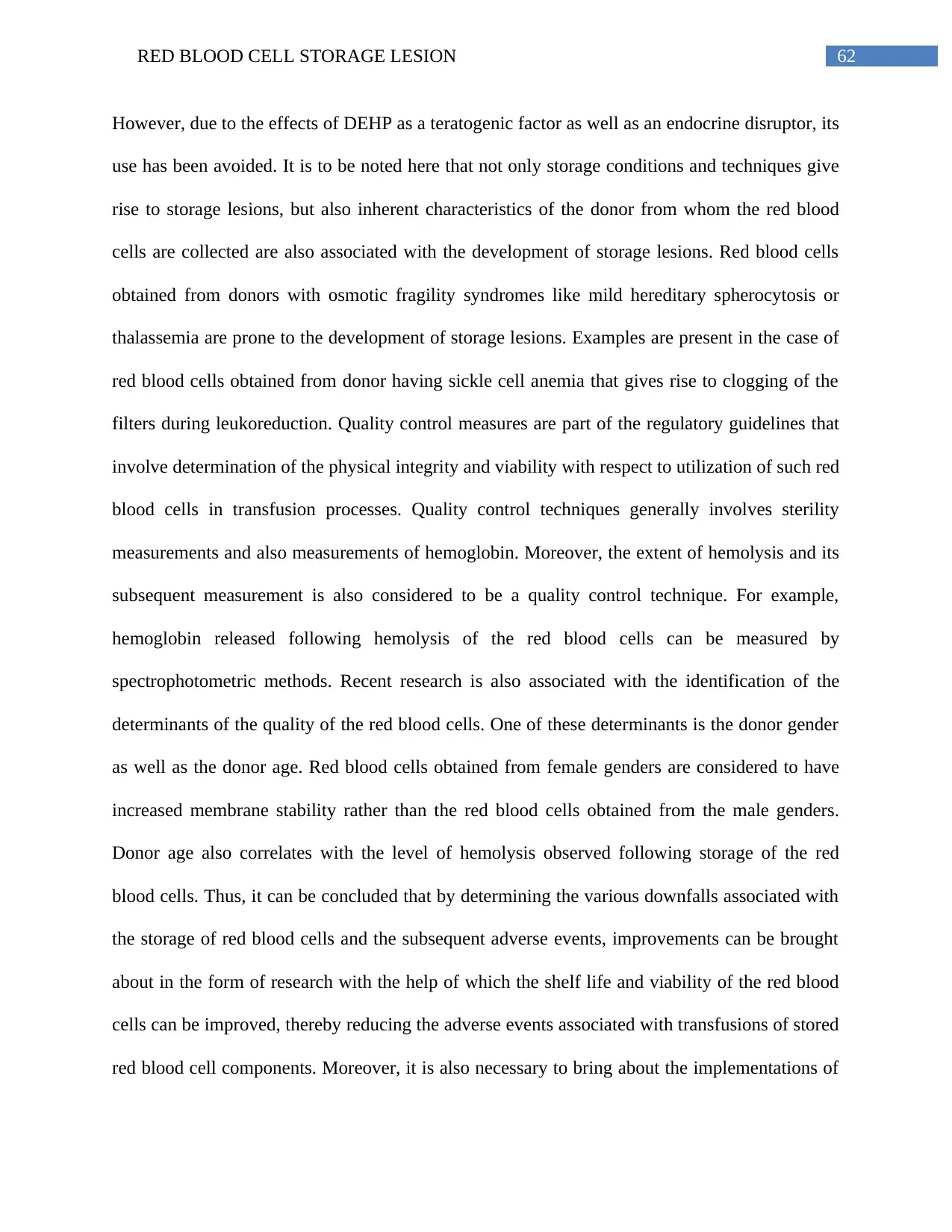
62RED BLOOD CELL STORAGE LESION
However, due to the effects of DEHP as a teratogenic factor as well as an endocrine disruptor, its
use has been avoided. It is to be noted here that not only storage conditions and techniques give
rise to storage lesions, but also inherent characteristics of the donor from whom the red blood
cells are collected are also associated with the development of storage lesions. Red blood cells
obtained from donors with osmotic fragility syndromes like mild hereditary spherocytosis or
thalassemia are prone to the development of storage lesions. Examples are present in the case of
red blood cells obtained from donor having sickle cell anemia that gives rise to clogging of the
filters during leukoreduction. Quality control measures are part of the regulatory guidelines that
involve determination of the physical integrity and viability with respect to utilization of such red
blood cells in transfusion processes. Quality control techniques generally involves sterility
measurements and also measurements of hemoglobin. Moreover, the extent of hemolysis and its
subsequent measurement is also considered to be a quality control technique. For example,
hemoglobin released following hemolysis of the red blood cells can be measured by
spectrophotometric methods. Recent research is also associated with the identification of the
determinants of the quality of the red blood cells. One of these determinants is the donor gender
as well as the donor age. Red blood cells obtained from female genders are considered to have
increased membrane stability rather than the red blood cells obtained from the male genders.
Donor age also correlates with the level of hemolysis observed following storage of the red
blood cells. Thus, it can be concluded that by determining the various downfalls associated with
the storage of red blood cells and the subsequent adverse events, improvements can be brought
about in the form of research with the help of which the shelf life and viability of the red blood
cells can be improved, thereby reducing the adverse events associated with transfusions of stored
red blood cell components. Moreover, it is also necessary to bring about the implementations of
However, due to the effects of DEHP as a teratogenic factor as well as an endocrine disruptor, its
use has been avoided. It is to be noted here that not only storage conditions and techniques give
rise to storage lesions, but also inherent characteristics of the donor from whom the red blood
cells are collected are also associated with the development of storage lesions. Red blood cells
obtained from donors with osmotic fragility syndromes like mild hereditary spherocytosis or
thalassemia are prone to the development of storage lesions. Examples are present in the case of
red blood cells obtained from donor having sickle cell anemia that gives rise to clogging of the
filters during leukoreduction. Quality control measures are part of the regulatory guidelines that
involve determination of the physical integrity and viability with respect to utilization of such red
blood cells in transfusion processes. Quality control techniques generally involves sterility
measurements and also measurements of hemoglobin. Moreover, the extent of hemolysis and its
subsequent measurement is also considered to be a quality control technique. For example,
hemoglobin released following hemolysis of the red blood cells can be measured by
spectrophotometric methods. Recent research is also associated with the identification of the
determinants of the quality of the red blood cells. One of these determinants is the donor gender
as well as the donor age. Red blood cells obtained from female genders are considered to have
increased membrane stability rather than the red blood cells obtained from the male genders.
Donor age also correlates with the level of hemolysis observed following storage of the red
blood cells. Thus, it can be concluded that by determining the various downfalls associated with
the storage of red blood cells and the subsequent adverse events, improvements can be brought
about in the form of research with the help of which the shelf life and viability of the red blood
cells can be improved, thereby reducing the adverse events associated with transfusions of stored
red blood cell components. Moreover, it is also necessary to bring about the implementations of
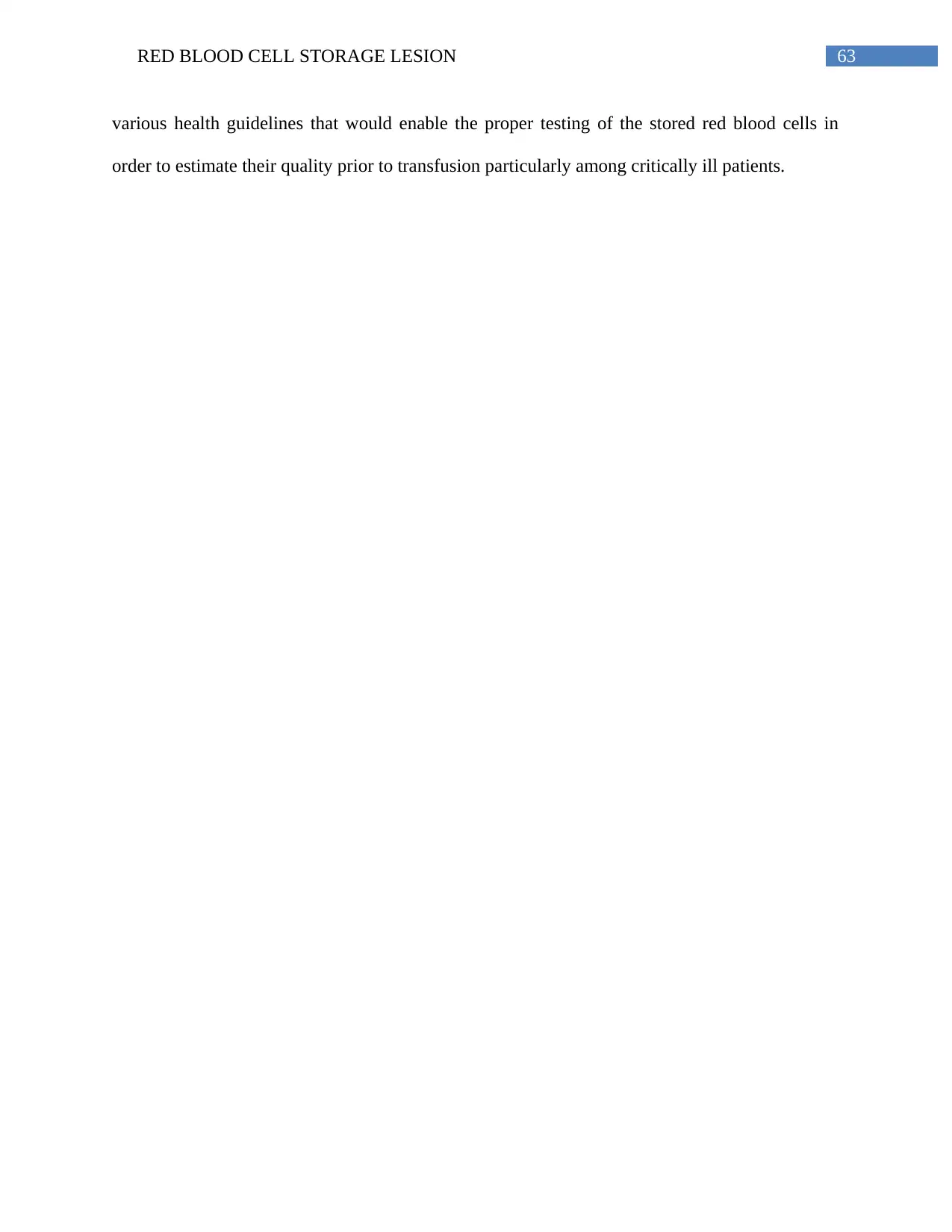
63RED BLOOD CELL STORAGE LESION
various health guidelines that would enable the proper testing of the stored red blood cells in
order to estimate their quality prior to transfusion particularly among critically ill patients.
various health guidelines that would enable the proper testing of the stored red blood cells in
order to estimate their quality prior to transfusion particularly among critically ill patients.
Secure Best Marks with AI Grader
Need help grading? Try our AI Grader for instant feedback on your assignments.
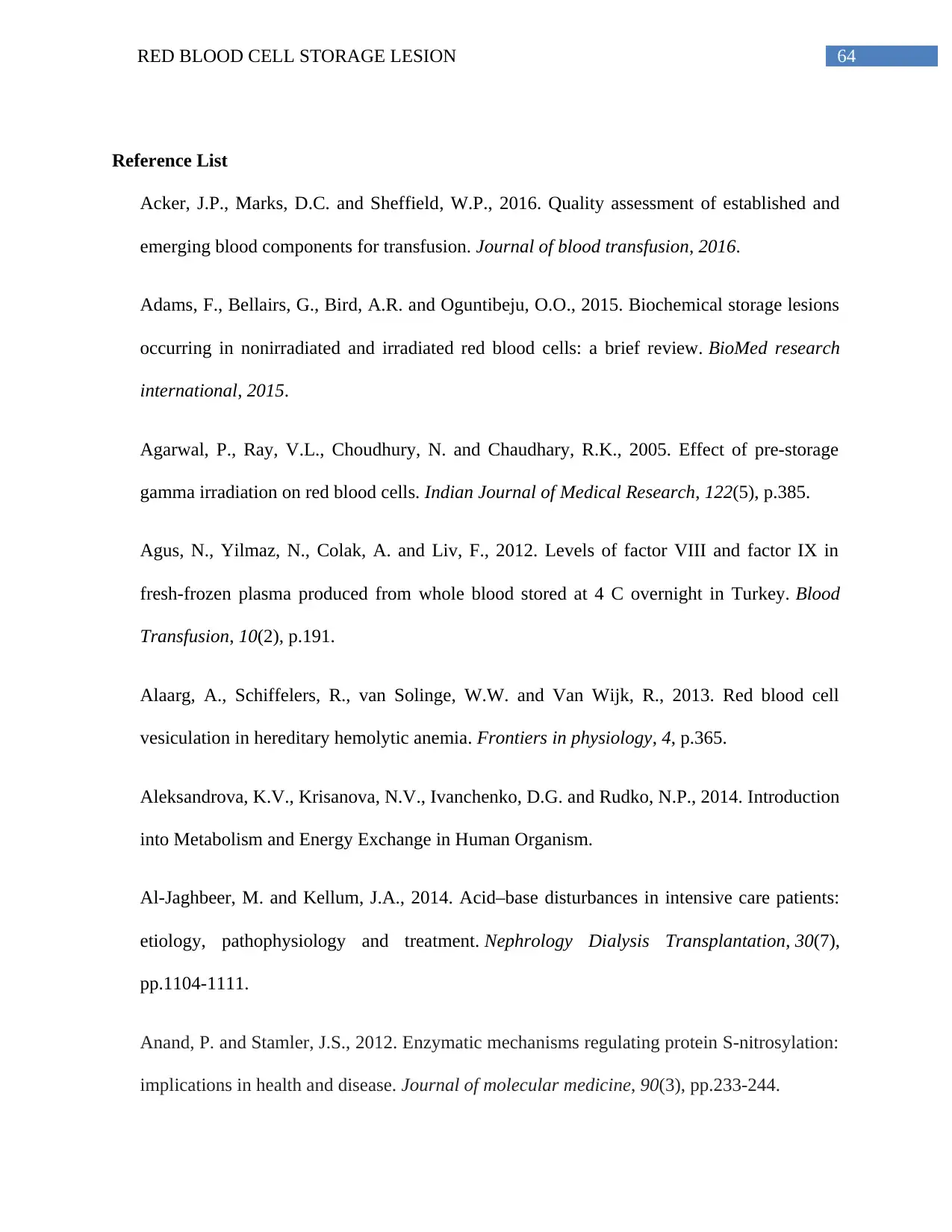
64RED BLOOD CELL STORAGE LESION
Reference List
Acker, J.P., Marks, D.C. and Sheffield, W.P., 2016. Quality assessment of established and
emerging blood components for transfusion. Journal of blood transfusion, 2016.
Adams, F., Bellairs, G., Bird, A.R. and Oguntibeju, O.O., 2015. Biochemical storage lesions
occurring in nonirradiated and irradiated red blood cells: a brief review. BioMed research
international, 2015.
Agarwal, P., Ray, V.L., Choudhury, N. and Chaudhary, R.K., 2005. Effect of pre-storage
gamma irradiation on red blood cells. Indian Journal of Medical Research, 122(5), p.385.
Agus, N., Yilmaz, N., Colak, A. and Liv, F., 2012. Levels of factor VIII and factor IX in
fresh-frozen plasma produced from whole blood stored at 4 C overnight in Turkey. Blood
Transfusion, 10(2), p.191.
Alaarg, A., Schiffelers, R., van Solinge, W.W. and Van Wijk, R., 2013. Red blood cell
vesiculation in hereditary hemolytic anemia. Frontiers in physiology, 4, p.365.
Aleksandrova, K.V., Krisanova, N.V., Ivanchenko, D.G. and Rudko, N.P., 2014. Introduction
into Metabolism and Energy Exchange in Human Organism.
Al-Jaghbeer, M. and Kellum, J.A., 2014. Acid–base disturbances in intensive care patients:
etiology, pathophysiology and treatment. Nephrology Dialysis Transplantation, 30(7),
pp.1104-1111.
Anand, P. and Stamler, J.S., 2012. Enzymatic mechanisms regulating protein S-nitrosylation:
implications in health and disease. Journal of molecular medicine, 90(3), pp.233-244.
Reference List
Acker, J.P., Marks, D.C. and Sheffield, W.P., 2016. Quality assessment of established and
emerging blood components for transfusion. Journal of blood transfusion, 2016.
Adams, F., Bellairs, G., Bird, A.R. and Oguntibeju, O.O., 2015. Biochemical storage lesions
occurring in nonirradiated and irradiated red blood cells: a brief review. BioMed research
international, 2015.
Agarwal, P., Ray, V.L., Choudhury, N. and Chaudhary, R.K., 2005. Effect of pre-storage
gamma irradiation on red blood cells. Indian Journal of Medical Research, 122(5), p.385.
Agus, N., Yilmaz, N., Colak, A. and Liv, F., 2012. Levels of factor VIII and factor IX in
fresh-frozen plasma produced from whole blood stored at 4 C overnight in Turkey. Blood
Transfusion, 10(2), p.191.
Alaarg, A., Schiffelers, R., van Solinge, W.W. and Van Wijk, R., 2013. Red blood cell
vesiculation in hereditary hemolytic anemia. Frontiers in physiology, 4, p.365.
Aleksandrova, K.V., Krisanova, N.V., Ivanchenko, D.G. and Rudko, N.P., 2014. Introduction
into Metabolism and Energy Exchange in Human Organism.
Al-Jaghbeer, M. and Kellum, J.A., 2014. Acid–base disturbances in intensive care patients:
etiology, pathophysiology and treatment. Nephrology Dialysis Transplantation, 30(7),
pp.1104-1111.
Anand, P. and Stamler, J.S., 2012. Enzymatic mechanisms regulating protein S-nitrosylation:
implications in health and disease. Journal of molecular medicine, 90(3), pp.233-244.
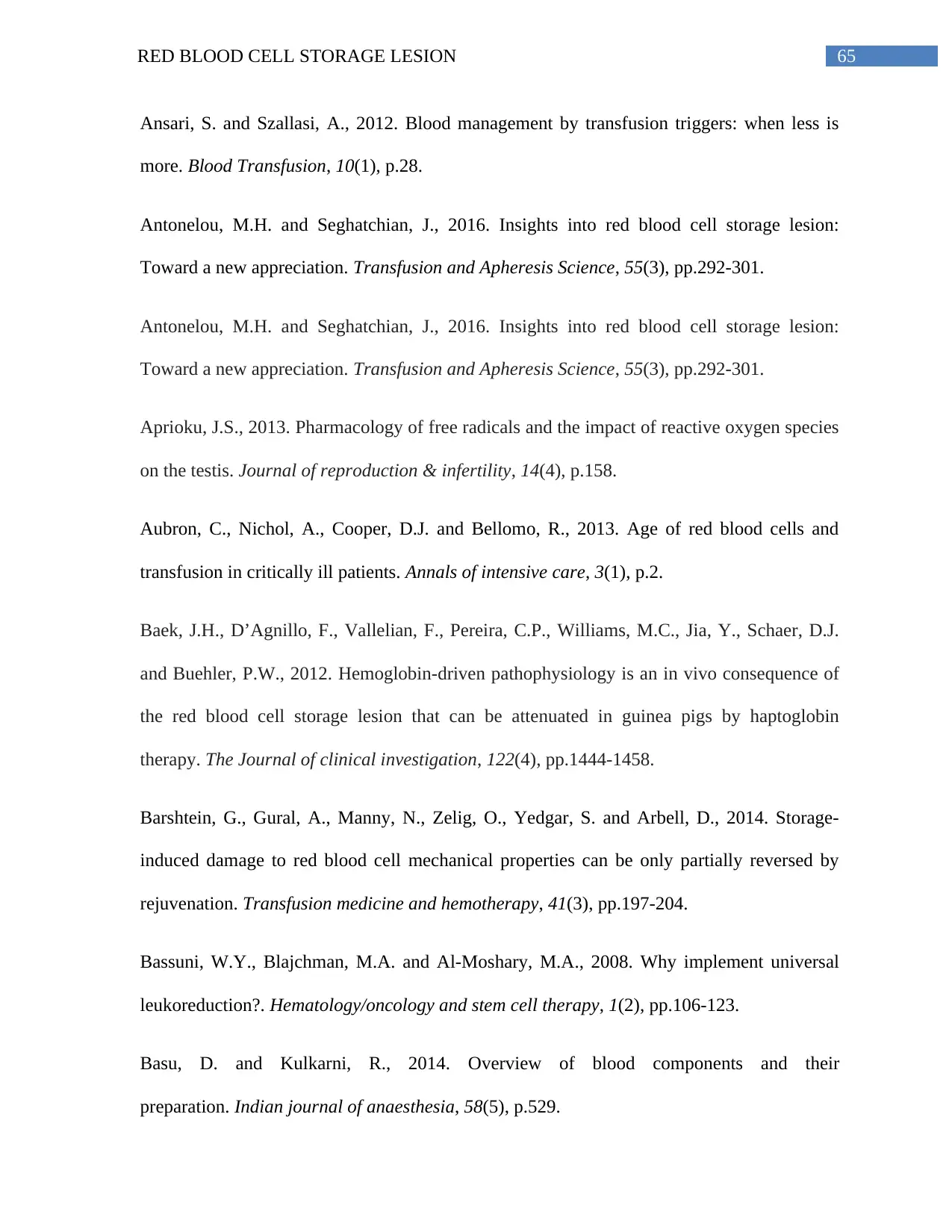
65RED BLOOD CELL STORAGE LESION
Ansari, S. and Szallasi, A., 2012. Blood management by transfusion triggers: when less is
more. Blood Transfusion, 10(1), p.28.
Antonelou, M.H. and Seghatchian, J., 2016. Insights into red blood cell storage lesion:
Toward a new appreciation. Transfusion and Apheresis Science, 55(3), pp.292-301.
Antonelou, M.H. and Seghatchian, J., 2016. Insights into red blood cell storage lesion:
Toward a new appreciation. Transfusion and Apheresis Science, 55(3), pp.292-301.
Aprioku, J.S., 2013. Pharmacology of free radicals and the impact of reactive oxygen species
on the testis. Journal of reproduction & infertility, 14(4), p.158.
Aubron, C., Nichol, A., Cooper, D.J. and Bellomo, R., 2013. Age of red blood cells and
transfusion in critically ill patients. Annals of intensive care, 3(1), p.2.
Baek, J.H., D’Agnillo, F., Vallelian, F., Pereira, C.P., Williams, M.C., Jia, Y., Schaer, D.J.
and Buehler, P.W., 2012. Hemoglobin-driven pathophysiology is an in vivo consequence of
the red blood cell storage lesion that can be attenuated in guinea pigs by haptoglobin
therapy. The Journal of clinical investigation, 122(4), pp.1444-1458.
Barshtein, G., Gural, A., Manny, N., Zelig, O., Yedgar, S. and Arbell, D., 2014. Storage-
induced damage to red blood cell mechanical properties can be only partially reversed by
rejuvenation. Transfusion medicine and hemotherapy, 41(3), pp.197-204.
Bassuni, W.Y., Blajchman, M.A. and Al-Moshary, M.A., 2008. Why implement universal
leukoreduction?. Hematology/oncology and stem cell therapy, 1(2), pp.106-123.
Basu, D. and Kulkarni, R., 2014. Overview of blood components and their
preparation. Indian journal of anaesthesia, 58(5), p.529.
Ansari, S. and Szallasi, A., 2012. Blood management by transfusion triggers: when less is
more. Blood Transfusion, 10(1), p.28.
Antonelou, M.H. and Seghatchian, J., 2016. Insights into red blood cell storage lesion:
Toward a new appreciation. Transfusion and Apheresis Science, 55(3), pp.292-301.
Antonelou, M.H. and Seghatchian, J., 2016. Insights into red blood cell storage lesion:
Toward a new appreciation. Transfusion and Apheresis Science, 55(3), pp.292-301.
Aprioku, J.S., 2013. Pharmacology of free radicals and the impact of reactive oxygen species
on the testis. Journal of reproduction & infertility, 14(4), p.158.
Aubron, C., Nichol, A., Cooper, D.J. and Bellomo, R., 2013. Age of red blood cells and
transfusion in critically ill patients. Annals of intensive care, 3(1), p.2.
Baek, J.H., D’Agnillo, F., Vallelian, F., Pereira, C.P., Williams, M.C., Jia, Y., Schaer, D.J.
and Buehler, P.W., 2012. Hemoglobin-driven pathophysiology is an in vivo consequence of
the red blood cell storage lesion that can be attenuated in guinea pigs by haptoglobin
therapy. The Journal of clinical investigation, 122(4), pp.1444-1458.
Barshtein, G., Gural, A., Manny, N., Zelig, O., Yedgar, S. and Arbell, D., 2014. Storage-
induced damage to red blood cell mechanical properties can be only partially reversed by
rejuvenation. Transfusion medicine and hemotherapy, 41(3), pp.197-204.
Bassuni, W.Y., Blajchman, M.A. and Al-Moshary, M.A., 2008. Why implement universal
leukoreduction?. Hematology/oncology and stem cell therapy, 1(2), pp.106-123.
Basu, D. and Kulkarni, R., 2014. Overview of blood components and their
preparation. Indian journal of anaesthesia, 58(5), p.529.
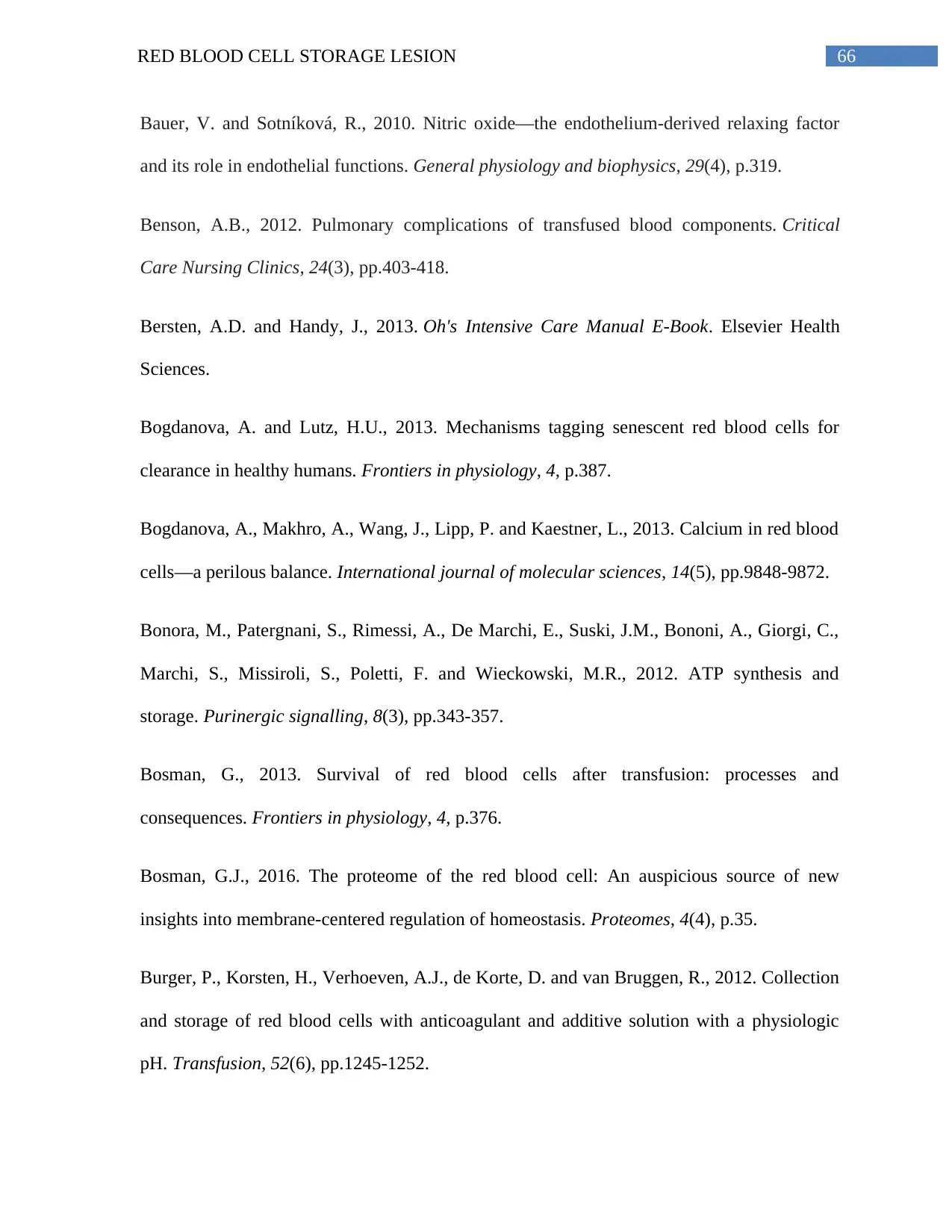
66RED BLOOD CELL STORAGE LESION
Bauer, V. and Sotníková, R., 2010. Nitric oxide—the endothelium-derived relaxing factor
and its role in endothelial functions. General physiology and biophysics, 29(4), p.319.
Benson, A.B., 2012. Pulmonary complications of transfused blood components. Critical
Care Nursing Clinics, 24(3), pp.403-418.
Bersten, A.D. and Handy, J., 2013. Oh's Intensive Care Manual E-Book. Elsevier Health
Sciences.
Bogdanova, A. and Lutz, H.U., 2013. Mechanisms tagging senescent red blood cells for
clearance in healthy humans. Frontiers in physiology, 4, p.387.
Bogdanova, A., Makhro, A., Wang, J., Lipp, P. and Kaestner, L., 2013. Calcium in red blood
cells—a perilous balance. International journal of molecular sciences, 14(5), pp.9848-9872.
Bonora, M., Patergnani, S., Rimessi, A., De Marchi, E., Suski, J.M., Bononi, A., Giorgi, C.,
Marchi, S., Missiroli, S., Poletti, F. and Wieckowski, M.R., 2012. ATP synthesis and
storage. Purinergic signalling, 8(3), pp.343-357.
Bosman, G., 2013. Survival of red blood cells after transfusion: processes and
consequences. Frontiers in physiology, 4, p.376.
Bosman, G.J., 2016. The proteome of the red blood cell: An auspicious source of new
insights into membrane-centered regulation of homeostasis. Proteomes, 4(4), p.35.
Burger, P., Korsten, H., Verhoeven, A.J., de Korte, D. and van Bruggen, R., 2012. Collection
and storage of red blood cells with anticoagulant and additive solution with a physiologic
pH. Transfusion, 52(6), pp.1245-1252.
Bauer, V. and Sotníková, R., 2010. Nitric oxide—the endothelium-derived relaxing factor
and its role in endothelial functions. General physiology and biophysics, 29(4), p.319.
Benson, A.B., 2012. Pulmonary complications of transfused blood components. Critical
Care Nursing Clinics, 24(3), pp.403-418.
Bersten, A.D. and Handy, J., 2013. Oh's Intensive Care Manual E-Book. Elsevier Health
Sciences.
Bogdanova, A. and Lutz, H.U., 2013. Mechanisms tagging senescent red blood cells for
clearance in healthy humans. Frontiers in physiology, 4, p.387.
Bogdanova, A., Makhro, A., Wang, J., Lipp, P. and Kaestner, L., 2013. Calcium in red blood
cells—a perilous balance. International journal of molecular sciences, 14(5), pp.9848-9872.
Bonora, M., Patergnani, S., Rimessi, A., De Marchi, E., Suski, J.M., Bononi, A., Giorgi, C.,
Marchi, S., Missiroli, S., Poletti, F. and Wieckowski, M.R., 2012. ATP synthesis and
storage. Purinergic signalling, 8(3), pp.343-357.
Bosman, G., 2013. Survival of red blood cells after transfusion: processes and
consequences. Frontiers in physiology, 4, p.376.
Bosman, G.J., 2016. The proteome of the red blood cell: An auspicious source of new
insights into membrane-centered regulation of homeostasis. Proteomes, 4(4), p.35.
Burger, P., Korsten, H., Verhoeven, A.J., de Korte, D. and van Bruggen, R., 2012. Collection
and storage of red blood cells with anticoagulant and additive solution with a physiologic
pH. Transfusion, 52(6), pp.1245-1252.
Paraphrase This Document
Need a fresh take? Get an instant paraphrase of this document with our AI Paraphraser
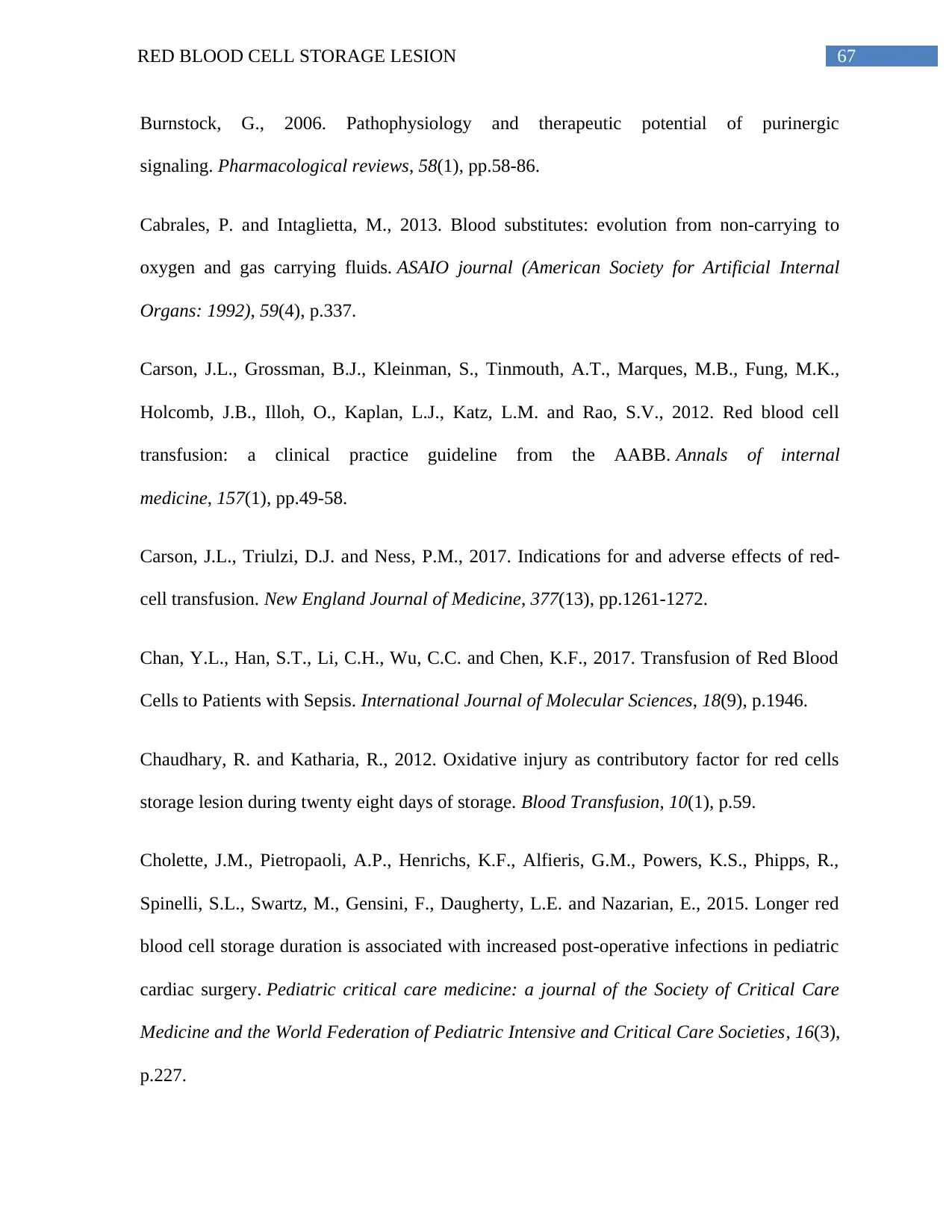
67RED BLOOD CELL STORAGE LESION
Burnstock, G., 2006. Pathophysiology and therapeutic potential of purinergic
signaling. Pharmacological reviews, 58(1), pp.58-86.
Cabrales, P. and Intaglietta, M., 2013. Blood substitutes: evolution from non-carrying to
oxygen and gas carrying fluids. ASAIO journal (American Society for Artificial Internal
Organs: 1992), 59(4), p.337.
Carson, J.L., Grossman, B.J., Kleinman, S., Tinmouth, A.T., Marques, M.B., Fung, M.K.,
Holcomb, J.B., Illoh, O., Kaplan, L.J., Katz, L.M. and Rao, S.V., 2012. Red blood cell
transfusion: a clinical practice guideline from the AABB. Annals of internal
medicine, 157(1), pp.49-58.
Carson, J.L., Triulzi, D.J. and Ness, P.M., 2017. Indications for and adverse effects of red-
cell transfusion. New England Journal of Medicine, 377(13), pp.1261-1272.
Chan, Y.L., Han, S.T., Li, C.H., Wu, C.C. and Chen, K.F., 2017. Transfusion of Red Blood
Cells to Patients with Sepsis. International Journal of Molecular Sciences, 18(9), p.1946.
Chaudhary, R. and Katharia, R., 2012. Oxidative injury as contributory factor for red cells
storage lesion during twenty eight days of storage. Blood Transfusion, 10(1), p.59.
Cholette, J.M., Pietropaoli, A.P., Henrichs, K.F., Alfieris, G.M., Powers, K.S., Phipps, R.,
Spinelli, S.L., Swartz, M., Gensini, F., Daugherty, L.E. and Nazarian, E., 2015. Longer red
blood cell storage duration is associated with increased post-operative infections in pediatric
cardiac surgery. Pediatric critical care medicine: a journal of the Society of Critical Care
Medicine and the World Federation of Pediatric Intensive and Critical Care Societies, 16(3),
p.227.
Burnstock, G., 2006. Pathophysiology and therapeutic potential of purinergic
signaling. Pharmacological reviews, 58(1), pp.58-86.
Cabrales, P. and Intaglietta, M., 2013. Blood substitutes: evolution from non-carrying to
oxygen and gas carrying fluids. ASAIO journal (American Society for Artificial Internal
Organs: 1992), 59(4), p.337.
Carson, J.L., Grossman, B.J., Kleinman, S., Tinmouth, A.T., Marques, M.B., Fung, M.K.,
Holcomb, J.B., Illoh, O., Kaplan, L.J., Katz, L.M. and Rao, S.V., 2012. Red blood cell
transfusion: a clinical practice guideline from the AABB. Annals of internal
medicine, 157(1), pp.49-58.
Carson, J.L., Triulzi, D.J. and Ness, P.M., 2017. Indications for and adverse effects of red-
cell transfusion. New England Journal of Medicine, 377(13), pp.1261-1272.
Chan, Y.L., Han, S.T., Li, C.H., Wu, C.C. and Chen, K.F., 2017. Transfusion of Red Blood
Cells to Patients with Sepsis. International Journal of Molecular Sciences, 18(9), p.1946.
Chaudhary, R. and Katharia, R., 2012. Oxidative injury as contributory factor for red cells
storage lesion during twenty eight days of storage. Blood Transfusion, 10(1), p.59.
Cholette, J.M., Pietropaoli, A.P., Henrichs, K.F., Alfieris, G.M., Powers, K.S., Phipps, R.,
Spinelli, S.L., Swartz, M., Gensini, F., Daugherty, L.E. and Nazarian, E., 2015. Longer red
blood cell storage duration is associated with increased post-operative infections in pediatric
cardiac surgery. Pediatric critical care medicine: a journal of the Society of Critical Care
Medicine and the World Federation of Pediatric Intensive and Critical Care Societies, 16(3),
p.227.
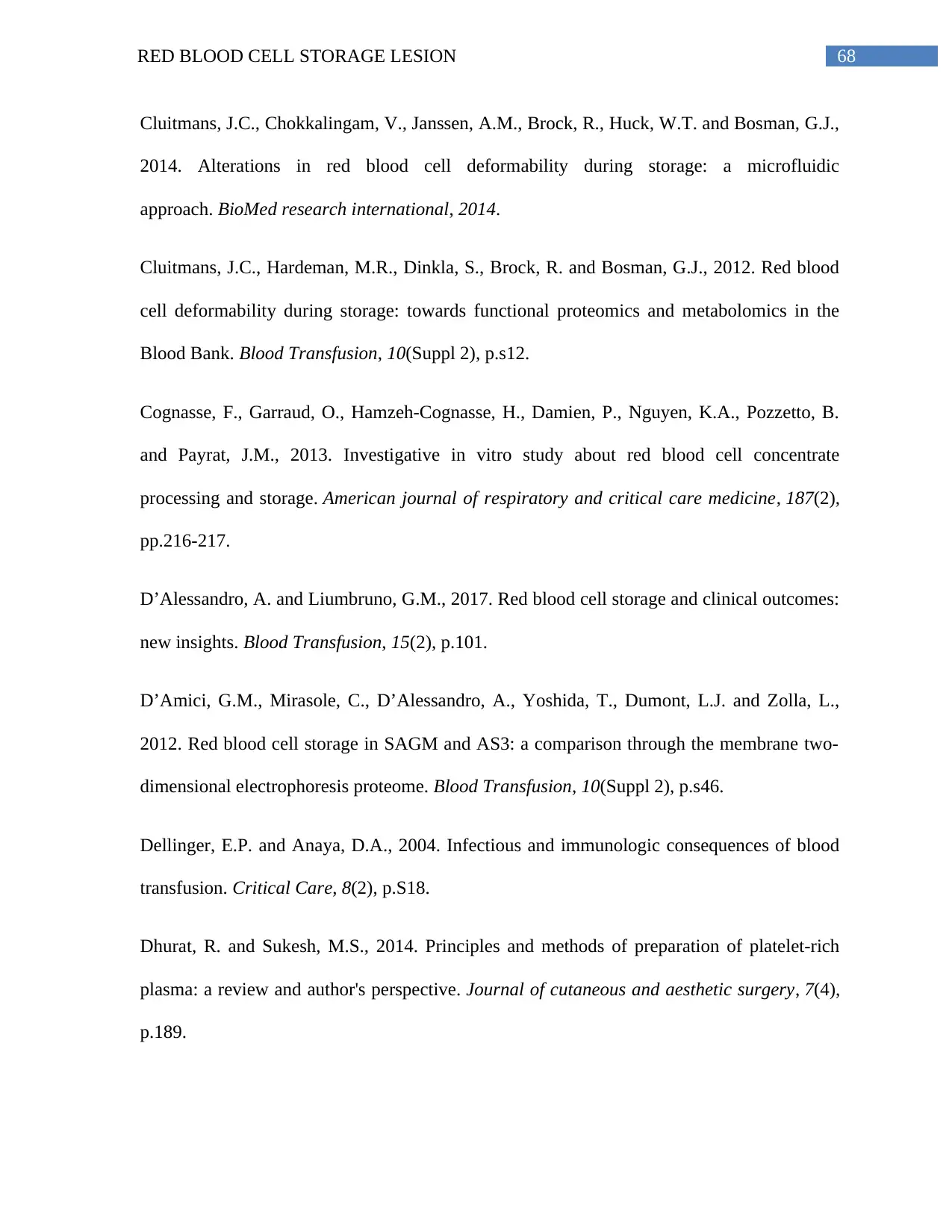
68RED BLOOD CELL STORAGE LESION
Cluitmans, J.C., Chokkalingam, V., Janssen, A.M., Brock, R., Huck, W.T. and Bosman, G.J.,
2014. Alterations in red blood cell deformability during storage: a microfluidic
approach. BioMed research international, 2014.
Cluitmans, J.C., Hardeman, M.R., Dinkla, S., Brock, R. and Bosman, G.J., 2012. Red blood
cell deformability during storage: towards functional proteomics and metabolomics in the
Blood Bank. Blood Transfusion, 10(Suppl 2), p.s12.
Cognasse, F., Garraud, O., Hamzeh-Cognasse, H., Damien, P., Nguyen, K.A., Pozzetto, B.
and Payrat, J.M., 2013. Investigative in vitro study about red blood cell concentrate
processing and storage. American journal of respiratory and critical care medicine, 187(2),
pp.216-217.
D’Alessandro, A. and Liumbruno, G.M., 2017. Red blood cell storage and clinical outcomes:
new insights. Blood Transfusion, 15(2), p.101.
D’Amici, G.M., Mirasole, C., D’Alessandro, A., Yoshida, T., Dumont, L.J. and Zolla, L.,
2012. Red blood cell storage in SAGM and AS3: a comparison through the membrane two-
dimensional electrophoresis proteome. Blood Transfusion, 10(Suppl 2), p.s46.
Dellinger, E.P. and Anaya, D.A., 2004. Infectious and immunologic consequences of blood
transfusion. Critical Care, 8(2), p.S18.
Dhurat, R. and Sukesh, M.S., 2014. Principles and methods of preparation of platelet-rich
plasma: a review and author's perspective. Journal of cutaneous and aesthetic surgery, 7(4),
p.189.
Cluitmans, J.C., Chokkalingam, V., Janssen, A.M., Brock, R., Huck, W.T. and Bosman, G.J.,
2014. Alterations in red blood cell deformability during storage: a microfluidic
approach. BioMed research international, 2014.
Cluitmans, J.C., Hardeman, M.R., Dinkla, S., Brock, R. and Bosman, G.J., 2012. Red blood
cell deformability during storage: towards functional proteomics and metabolomics in the
Blood Bank. Blood Transfusion, 10(Suppl 2), p.s12.
Cognasse, F., Garraud, O., Hamzeh-Cognasse, H., Damien, P., Nguyen, K.A., Pozzetto, B.
and Payrat, J.M., 2013. Investigative in vitro study about red blood cell concentrate
processing and storage. American journal of respiratory and critical care medicine, 187(2),
pp.216-217.
D’Alessandro, A. and Liumbruno, G.M., 2017. Red blood cell storage and clinical outcomes:
new insights. Blood Transfusion, 15(2), p.101.
D’Amici, G.M., Mirasole, C., D’Alessandro, A., Yoshida, T., Dumont, L.J. and Zolla, L.,
2012. Red blood cell storage in SAGM and AS3: a comparison through the membrane two-
dimensional electrophoresis proteome. Blood Transfusion, 10(Suppl 2), p.s46.
Dellinger, E.P. and Anaya, D.A., 2004. Infectious and immunologic consequences of blood
transfusion. Critical Care, 8(2), p.S18.
Dhurat, R. and Sukesh, M.S., 2014. Principles and methods of preparation of platelet-rich
plasma: a review and author's perspective. Journal of cutaneous and aesthetic surgery, 7(4),
p.189.
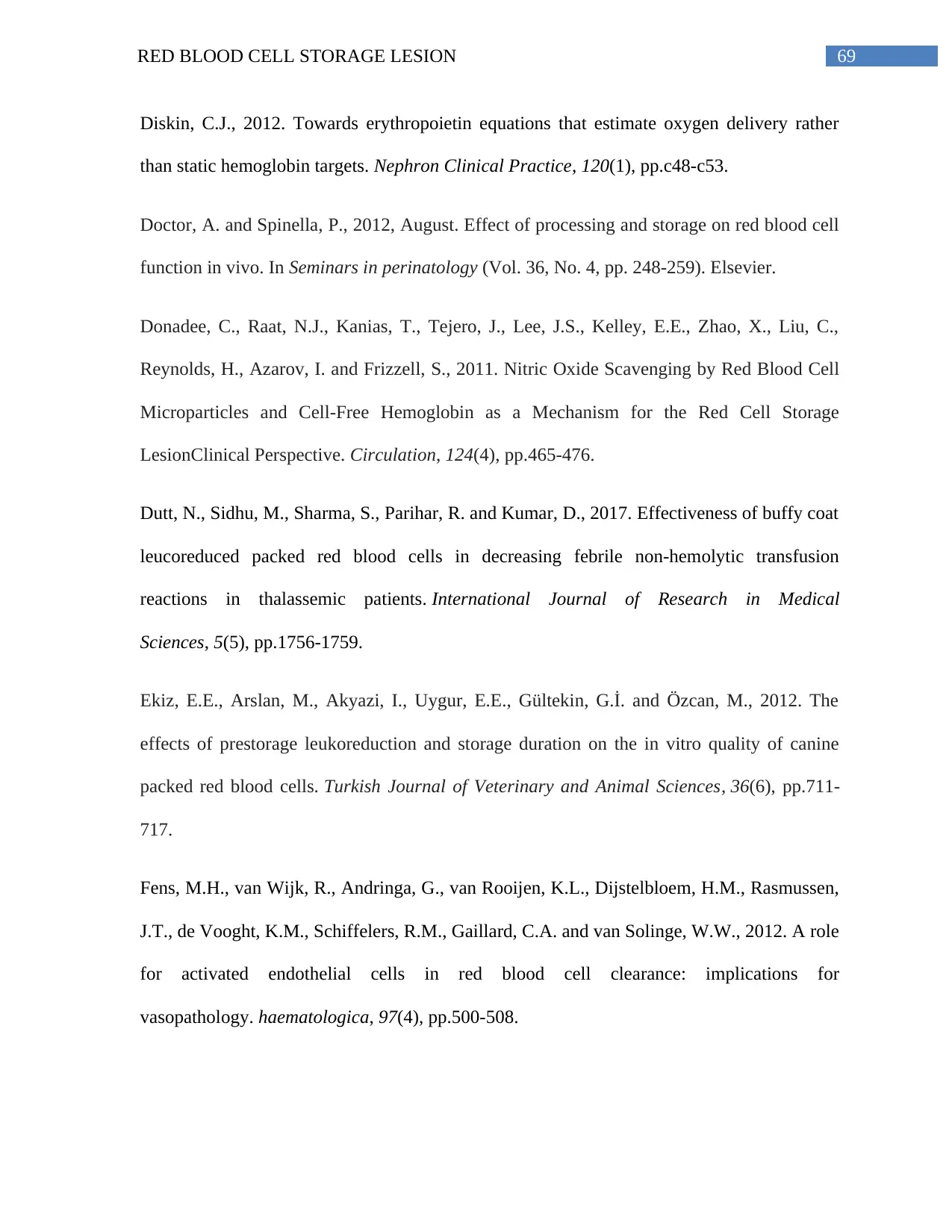
69RED BLOOD CELL STORAGE LESION
Diskin, C.J., 2012. Towards erythropoietin equations that estimate oxygen delivery rather
than static hemoglobin targets. Nephron Clinical Practice, 120(1), pp.c48-c53.
Doctor, A. and Spinella, P., 2012, August. Effect of processing and storage on red blood cell
function in vivo. In Seminars in perinatology (Vol. 36, No. 4, pp. 248-259). Elsevier.
Donadee, C., Raat, N.J., Kanias, T., Tejero, J., Lee, J.S., Kelley, E.E., Zhao, X., Liu, C.,
Reynolds, H., Azarov, I. and Frizzell, S., 2011. Nitric Oxide Scavenging by Red Blood Cell
Microparticles and Cell-Free Hemoglobin as a Mechanism for the Red Cell Storage
LesionClinical Perspective. Circulation, 124(4), pp.465-476.
Dutt, N., Sidhu, M., Sharma, S., Parihar, R. and Kumar, D., 2017. Effectiveness of buffy coat
leucoreduced packed red blood cells in decreasing febrile non-hemolytic transfusion
reactions in thalassemic patients. International Journal of Research in Medical
Sciences, 5(5), pp.1756-1759.
Ekiz, E.E., Arslan, M., Akyazi, I., Uygur, E.E., Gültekin, G.İ. and Özcan, M., 2012. The
effects of prestorage leukoreduction and storage duration on the in vitro quality of canine
packed red blood cells. Turkish Journal of Veterinary and Animal Sciences, 36(6), pp.711-
717.
Fens, M.H., van Wijk, R., Andringa, G., van Rooijen, K.L., Dijstelbloem, H.M., Rasmussen,
J.T., de Vooght, K.M., Schiffelers, R.M., Gaillard, C.A. and van Solinge, W.W., 2012. A role
for activated endothelial cells in red blood cell clearance: implications for
vasopathology. haematologica, 97(4), pp.500-508.
Diskin, C.J., 2012. Towards erythropoietin equations that estimate oxygen delivery rather
than static hemoglobin targets. Nephron Clinical Practice, 120(1), pp.c48-c53.
Doctor, A. and Spinella, P., 2012, August. Effect of processing and storage on red blood cell
function in vivo. In Seminars in perinatology (Vol. 36, No. 4, pp. 248-259). Elsevier.
Donadee, C., Raat, N.J., Kanias, T., Tejero, J., Lee, J.S., Kelley, E.E., Zhao, X., Liu, C.,
Reynolds, H., Azarov, I. and Frizzell, S., 2011. Nitric Oxide Scavenging by Red Blood Cell
Microparticles and Cell-Free Hemoglobin as a Mechanism for the Red Cell Storage
LesionClinical Perspective. Circulation, 124(4), pp.465-476.
Dutt, N., Sidhu, M., Sharma, S., Parihar, R. and Kumar, D., 2017. Effectiveness of buffy coat
leucoreduced packed red blood cells in decreasing febrile non-hemolytic transfusion
reactions in thalassemic patients. International Journal of Research in Medical
Sciences, 5(5), pp.1756-1759.
Ekiz, E.E., Arslan, M., Akyazi, I., Uygur, E.E., Gültekin, G.İ. and Özcan, M., 2012. The
effects of prestorage leukoreduction and storage duration on the in vitro quality of canine
packed red blood cells. Turkish Journal of Veterinary and Animal Sciences, 36(6), pp.711-
717.
Fens, M.H., van Wijk, R., Andringa, G., van Rooijen, K.L., Dijstelbloem, H.M., Rasmussen,
J.T., de Vooght, K.M., Schiffelers, R.M., Gaillard, C.A. and van Solinge, W.W., 2012. A role
for activated endothelial cells in red blood cell clearance: implications for
vasopathology. haematologica, 97(4), pp.500-508.
Secure Best Marks with AI Grader
Need help grading? Try our AI Grader for instant feedback on your assignments.
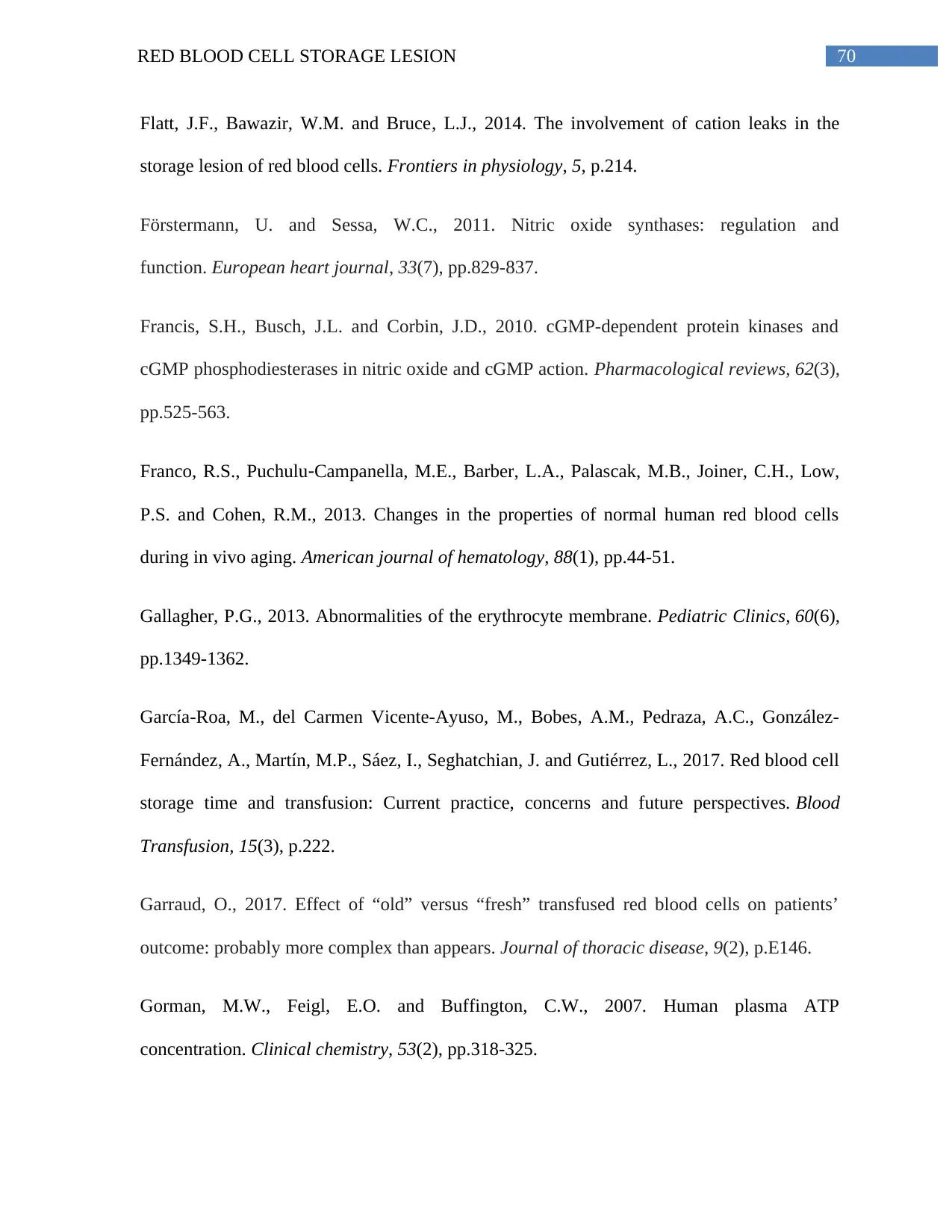
70RED BLOOD CELL STORAGE LESION
Flatt, J.F., Bawazir, W.M. and Bruce, L.J., 2014. The involvement of cation leaks in the
storage lesion of red blood cells. Frontiers in physiology, 5, p.214.
Förstermann, U. and Sessa, W.C., 2011. Nitric oxide synthases: regulation and
function. European heart journal, 33(7), pp.829-837.
Francis, S.H., Busch, J.L. and Corbin, J.D., 2010. cGMP-dependent protein kinases and
cGMP phosphodiesterases in nitric oxide and cGMP action. Pharmacological reviews, 62(3),
pp.525-563.
Franco, R.S., Puchulu‐Campanella, M.E., Barber, L.A., Palascak, M.B., Joiner, C.H., Low,
P.S. and Cohen, R.M., 2013. Changes in the properties of normal human red blood cells
during in vivo aging. American journal of hematology, 88(1), pp.44-51.
Gallagher, P.G., 2013. Abnormalities of the erythrocyte membrane. Pediatric Clinics, 60(6),
pp.1349-1362.
García-Roa, M., del Carmen Vicente-Ayuso, M., Bobes, A.M., Pedraza, A.C., González-
Fernández, A., Martín, M.P., Sáez, I., Seghatchian, J. and Gutiérrez, L., 2017. Red blood cell
storage time and transfusion: Current practice, concerns and future perspectives. Blood
Transfusion, 15(3), p.222.
Garraud, O., 2017. Effect of “old” versus “fresh” transfused red blood cells on patients’
outcome: probably more complex than appears. Journal of thoracic disease, 9(2), p.E146.
Gorman, M.W., Feigl, E.O. and Buffington, C.W., 2007. Human plasma ATP
concentration. Clinical chemistry, 53(2), pp.318-325.
Flatt, J.F., Bawazir, W.M. and Bruce, L.J., 2014. The involvement of cation leaks in the
storage lesion of red blood cells. Frontiers in physiology, 5, p.214.
Förstermann, U. and Sessa, W.C., 2011. Nitric oxide synthases: regulation and
function. European heart journal, 33(7), pp.829-837.
Francis, S.H., Busch, J.L. and Corbin, J.D., 2010. cGMP-dependent protein kinases and
cGMP phosphodiesterases in nitric oxide and cGMP action. Pharmacological reviews, 62(3),
pp.525-563.
Franco, R.S., Puchulu‐Campanella, M.E., Barber, L.A., Palascak, M.B., Joiner, C.H., Low,
P.S. and Cohen, R.M., 2013. Changes in the properties of normal human red blood cells
during in vivo aging. American journal of hematology, 88(1), pp.44-51.
Gallagher, P.G., 2013. Abnormalities of the erythrocyte membrane. Pediatric Clinics, 60(6),
pp.1349-1362.
García-Roa, M., del Carmen Vicente-Ayuso, M., Bobes, A.M., Pedraza, A.C., González-
Fernández, A., Martín, M.P., Sáez, I., Seghatchian, J. and Gutiérrez, L., 2017. Red blood cell
storage time and transfusion: Current practice, concerns and future perspectives. Blood
Transfusion, 15(3), p.222.
Garraud, O., 2017. Effect of “old” versus “fresh” transfused red blood cells on patients’
outcome: probably more complex than appears. Journal of thoracic disease, 9(2), p.E146.
Gorman, M.W., Feigl, E.O. and Buffington, C.W., 2007. Human plasma ATP
concentration. Clinical chemistry, 53(2), pp.318-325.
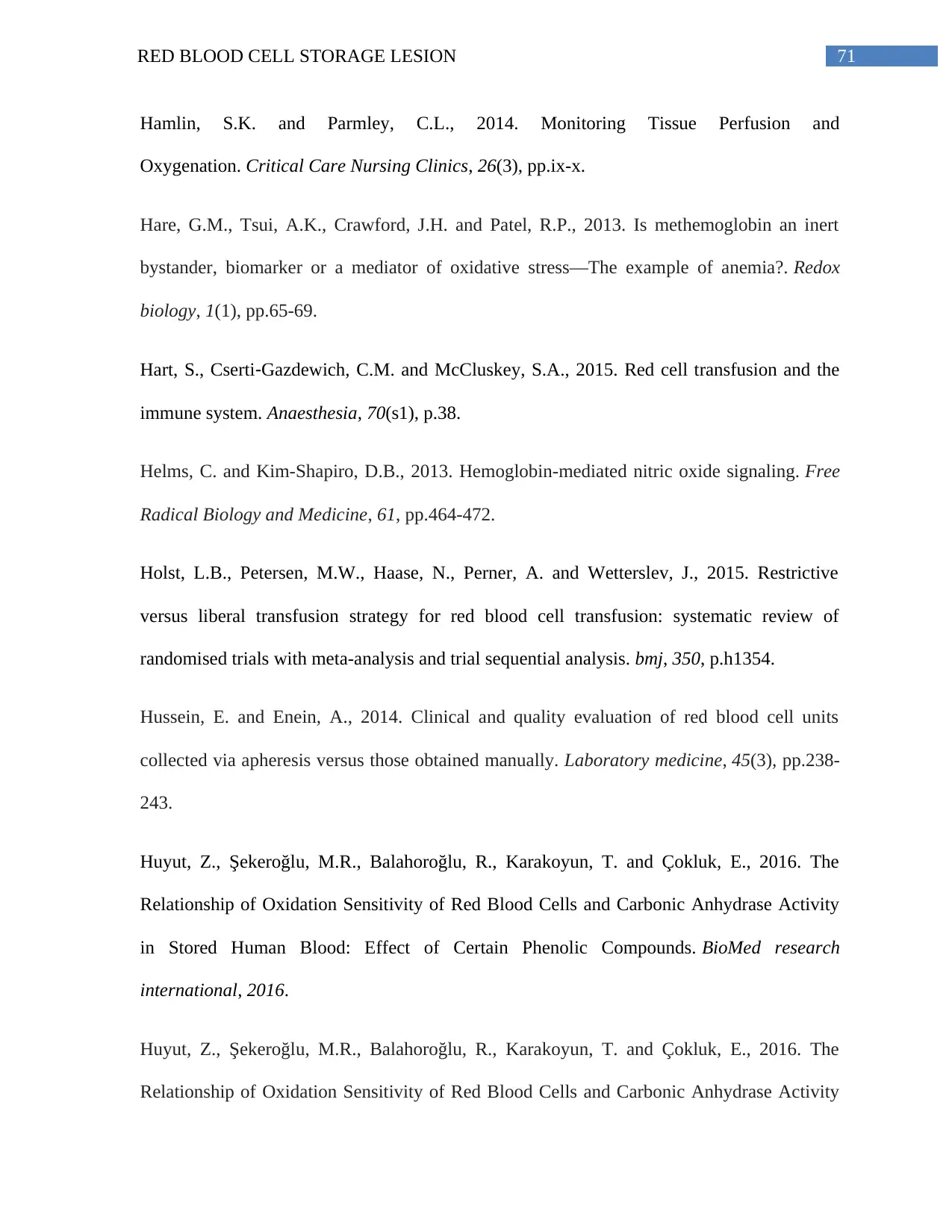
71RED BLOOD CELL STORAGE LESION
Hamlin, S.K. and Parmley, C.L., 2014. Monitoring Tissue Perfusion and
Oxygenation. Critical Care Nursing Clinics, 26(3), pp.ix-x.
Hare, G.M., Tsui, A.K., Crawford, J.H. and Patel, R.P., 2013. Is methemoglobin an inert
bystander, biomarker or a mediator of oxidative stress—The example of anemia?. Redox
biology, 1(1), pp.65-69.
Hart, S., Cserti‐Gazdewich, C.M. and McCluskey, S.A., 2015. Red cell transfusion and the
immune system. Anaesthesia, 70(s1), p.38.
Helms, C. and Kim-Shapiro, D.B., 2013. Hemoglobin-mediated nitric oxide signaling. Free
Radical Biology and Medicine, 61, pp.464-472.
Holst, L.B., Petersen, M.W., Haase, N., Perner, A. and Wetterslev, J., 2015. Restrictive
versus liberal transfusion strategy for red blood cell transfusion: systematic review of
randomised trials with meta-analysis and trial sequential analysis. bmj, 350, p.h1354.
Hussein, E. and Enein, A., 2014. Clinical and quality evaluation of red blood cell units
collected via apheresis versus those obtained manually. Laboratory medicine, 45(3), pp.238-
243.
Huyut, Z., Şekeroğlu, M.R., Balahoroğlu, R., Karakoyun, T. and Çokluk, E., 2016. The
Relationship of Oxidation Sensitivity of Red Blood Cells and Carbonic Anhydrase Activity
in Stored Human Blood: Effect of Certain Phenolic Compounds. BioMed research
international, 2016.
Huyut, Z., Şekeroğlu, M.R., Balahoroğlu, R., Karakoyun, T. and Çokluk, E., 2016. The
Relationship of Oxidation Sensitivity of Red Blood Cells and Carbonic Anhydrase Activity
Hamlin, S.K. and Parmley, C.L., 2014. Monitoring Tissue Perfusion and
Oxygenation. Critical Care Nursing Clinics, 26(3), pp.ix-x.
Hare, G.M., Tsui, A.K., Crawford, J.H. and Patel, R.P., 2013. Is methemoglobin an inert
bystander, biomarker or a mediator of oxidative stress—The example of anemia?. Redox
biology, 1(1), pp.65-69.
Hart, S., Cserti‐Gazdewich, C.M. and McCluskey, S.A., 2015. Red cell transfusion and the
immune system. Anaesthesia, 70(s1), p.38.
Helms, C. and Kim-Shapiro, D.B., 2013. Hemoglobin-mediated nitric oxide signaling. Free
Radical Biology and Medicine, 61, pp.464-472.
Holst, L.B., Petersen, M.W., Haase, N., Perner, A. and Wetterslev, J., 2015. Restrictive
versus liberal transfusion strategy for red blood cell transfusion: systematic review of
randomised trials with meta-analysis and trial sequential analysis. bmj, 350, p.h1354.
Hussein, E. and Enein, A., 2014. Clinical and quality evaluation of red blood cell units
collected via apheresis versus those obtained manually. Laboratory medicine, 45(3), pp.238-
243.
Huyut, Z., Şekeroğlu, M.R., Balahoroğlu, R., Karakoyun, T. and Çokluk, E., 2016. The
Relationship of Oxidation Sensitivity of Red Blood Cells and Carbonic Anhydrase Activity
in Stored Human Blood: Effect of Certain Phenolic Compounds. BioMed research
international, 2016.
Huyut, Z., Şekeroğlu, M.R., Balahoroğlu, R., Karakoyun, T. and Çokluk, E., 2016. The
Relationship of Oxidation Sensitivity of Red Blood Cells and Carbonic Anhydrase Activity
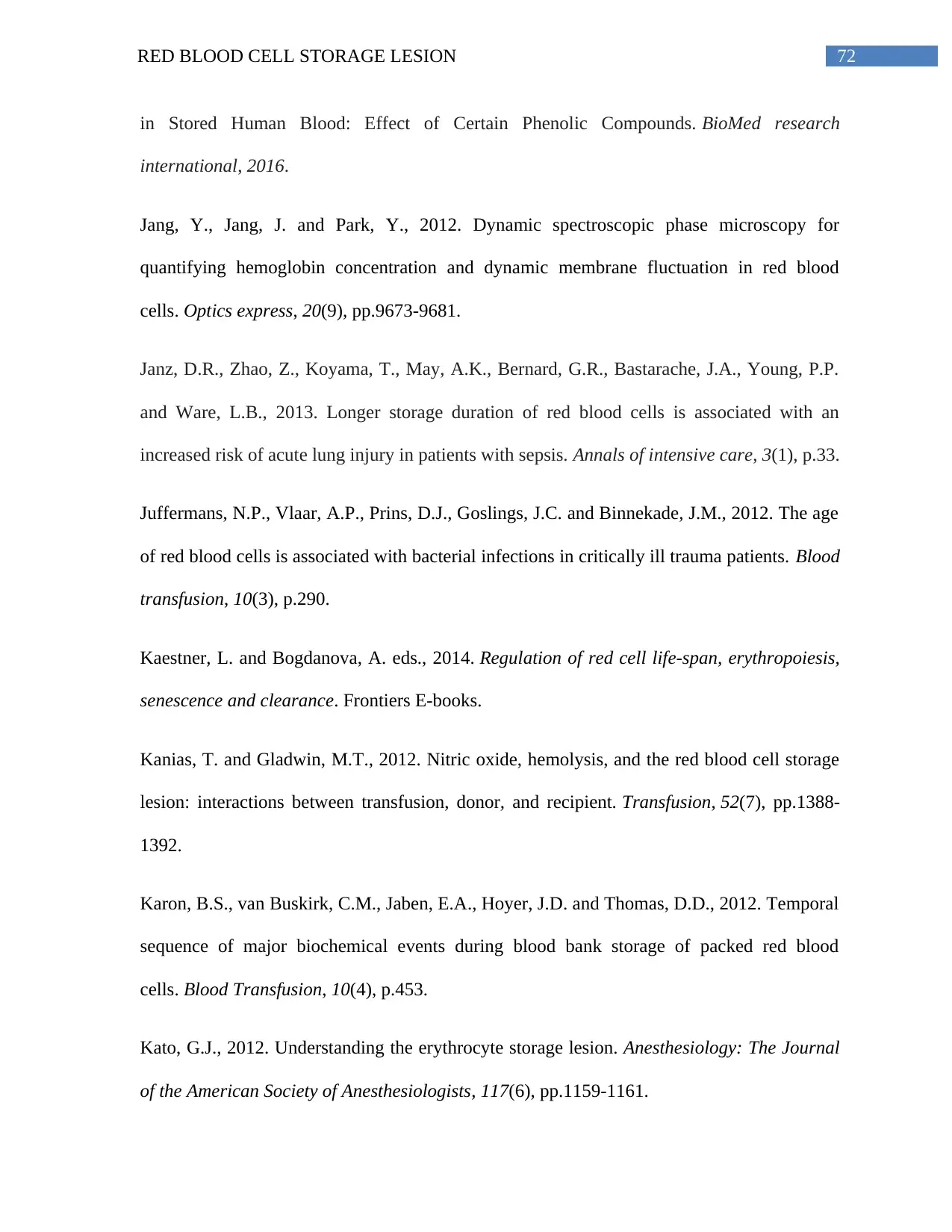
72RED BLOOD CELL STORAGE LESION
in Stored Human Blood: Effect of Certain Phenolic Compounds. BioMed research
international, 2016.
Jang, Y., Jang, J. and Park, Y., 2012. Dynamic spectroscopic phase microscopy for
quantifying hemoglobin concentration and dynamic membrane fluctuation in red blood
cells. Optics express, 20(9), pp.9673-9681.
Janz, D.R., Zhao, Z., Koyama, T., May, A.K., Bernard, G.R., Bastarache, J.A., Young, P.P.
and Ware, L.B., 2013. Longer storage duration of red blood cells is associated with an
increased risk of acute lung injury in patients with sepsis. Annals of intensive care, 3(1), p.33.
Juffermans, N.P., Vlaar, A.P., Prins, D.J., Goslings, J.C. and Binnekade, J.M., 2012. The age
of red blood cells is associated with bacterial infections in critically ill trauma patients. Blood
transfusion, 10(3), p.290.
Kaestner, L. and Bogdanova, A. eds., 2014. Regulation of red cell life-span, erythropoiesis,
senescence and clearance. Frontiers E-books.
Kanias, T. and Gladwin, M.T., 2012. Nitric oxide, hemolysis, and the red blood cell storage
lesion: interactions between transfusion, donor, and recipient. Transfusion, 52(7), pp.1388-
1392.
Karon, B.S., van Buskirk, C.M., Jaben, E.A., Hoyer, J.D. and Thomas, D.D., 2012. Temporal
sequence of major biochemical events during blood bank storage of packed red blood
cells. Blood Transfusion, 10(4), p.453.
Kato, G.J., 2012. Understanding the erythrocyte storage lesion. Anesthesiology: The Journal
of the American Society of Anesthesiologists, 117(6), pp.1159-1161.
in Stored Human Blood: Effect of Certain Phenolic Compounds. BioMed research
international, 2016.
Jang, Y., Jang, J. and Park, Y., 2012. Dynamic spectroscopic phase microscopy for
quantifying hemoglobin concentration and dynamic membrane fluctuation in red blood
cells. Optics express, 20(9), pp.9673-9681.
Janz, D.R., Zhao, Z., Koyama, T., May, A.K., Bernard, G.R., Bastarache, J.A., Young, P.P.
and Ware, L.B., 2013. Longer storage duration of red blood cells is associated with an
increased risk of acute lung injury in patients with sepsis. Annals of intensive care, 3(1), p.33.
Juffermans, N.P., Vlaar, A.P., Prins, D.J., Goslings, J.C. and Binnekade, J.M., 2012. The age
of red blood cells is associated with bacterial infections in critically ill trauma patients. Blood
transfusion, 10(3), p.290.
Kaestner, L. and Bogdanova, A. eds., 2014. Regulation of red cell life-span, erythropoiesis,
senescence and clearance. Frontiers E-books.
Kanias, T. and Gladwin, M.T., 2012. Nitric oxide, hemolysis, and the red blood cell storage
lesion: interactions between transfusion, donor, and recipient. Transfusion, 52(7), pp.1388-
1392.
Karon, B.S., van Buskirk, C.M., Jaben, E.A., Hoyer, J.D. and Thomas, D.D., 2012. Temporal
sequence of major biochemical events during blood bank storage of packed red blood
cells. Blood Transfusion, 10(4), p.453.
Kato, G.J., 2012. Understanding the erythrocyte storage lesion. Anesthesiology: The Journal
of the American Society of Anesthesiologists, 117(6), pp.1159-1161.
Paraphrase This Document
Need a fresh take? Get an instant paraphrase of this document with our AI Paraphraser
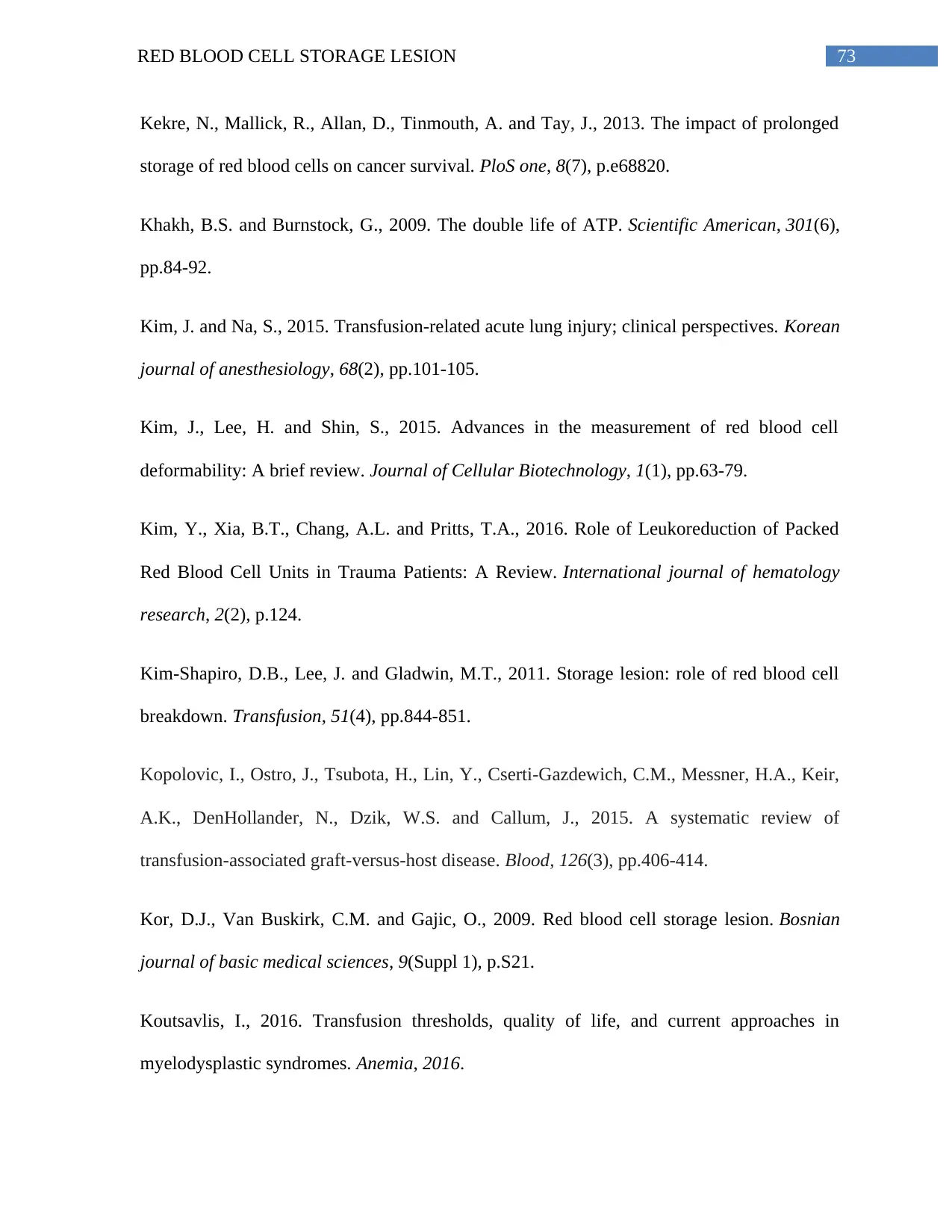
73RED BLOOD CELL STORAGE LESION
Kekre, N., Mallick, R., Allan, D., Tinmouth, A. and Tay, J., 2013. The impact of prolonged
storage of red blood cells on cancer survival. PloS one, 8(7), p.e68820.
Khakh, B.S. and Burnstock, G., 2009. The double life of ATP. Scientific American, 301(6),
pp.84-92.
Kim, J. and Na, S., 2015. Transfusion-related acute lung injury; clinical perspectives. Korean
journal of anesthesiology, 68(2), pp.101-105.
Kim, J., Lee, H. and Shin, S., 2015. Advances in the measurement of red blood cell
deformability: A brief review. Journal of Cellular Biotechnology, 1(1), pp.63-79.
Kim, Y., Xia, B.T., Chang, A.L. and Pritts, T.A., 2016. Role of Leukoreduction of Packed
Red Blood Cell Units in Trauma Patients: A Review. International journal of hematology
research, 2(2), p.124.
Kim‐Shapiro, D.B., Lee, J. and Gladwin, M.T., 2011. Storage lesion: role of red blood cell
breakdown. Transfusion, 51(4), pp.844-851.
Kopolovic, I., Ostro, J., Tsubota, H., Lin, Y., Cserti-Gazdewich, C.M., Messner, H.A., Keir,
A.K., DenHollander, N., Dzik, W.S. and Callum, J., 2015. A systematic review of
transfusion-associated graft-versus-host disease. Blood, 126(3), pp.406-414.
Kor, D.J., Van Buskirk, C.M. and Gajic, O., 2009. Red blood cell storage lesion. Bosnian
journal of basic medical sciences, 9(Suppl 1), p.S21.
Koutsavlis, I., 2016. Transfusion thresholds, quality of life, and current approaches in
myelodysplastic syndromes. Anemia, 2016.
Kekre, N., Mallick, R., Allan, D., Tinmouth, A. and Tay, J., 2013. The impact of prolonged
storage of red blood cells on cancer survival. PloS one, 8(7), p.e68820.
Khakh, B.S. and Burnstock, G., 2009. The double life of ATP. Scientific American, 301(6),
pp.84-92.
Kim, J. and Na, S., 2015. Transfusion-related acute lung injury; clinical perspectives. Korean
journal of anesthesiology, 68(2), pp.101-105.
Kim, J., Lee, H. and Shin, S., 2015. Advances in the measurement of red blood cell
deformability: A brief review. Journal of Cellular Biotechnology, 1(1), pp.63-79.
Kim, Y., Xia, B.T., Chang, A.L. and Pritts, T.A., 2016. Role of Leukoreduction of Packed
Red Blood Cell Units in Trauma Patients: A Review. International journal of hematology
research, 2(2), p.124.
Kim‐Shapiro, D.B., Lee, J. and Gladwin, M.T., 2011. Storage lesion: role of red blood cell
breakdown. Transfusion, 51(4), pp.844-851.
Kopolovic, I., Ostro, J., Tsubota, H., Lin, Y., Cserti-Gazdewich, C.M., Messner, H.A., Keir,
A.K., DenHollander, N., Dzik, W.S. and Callum, J., 2015. A systematic review of
transfusion-associated graft-versus-host disease. Blood, 126(3), pp.406-414.
Kor, D.J., Van Buskirk, C.M. and Gajic, O., 2009. Red blood cell storage lesion. Bosnian
journal of basic medical sciences, 9(Suppl 1), p.S21.
Koutsavlis, I., 2016. Transfusion thresholds, quality of life, and current approaches in
myelodysplastic syndromes. Anemia, 2016.
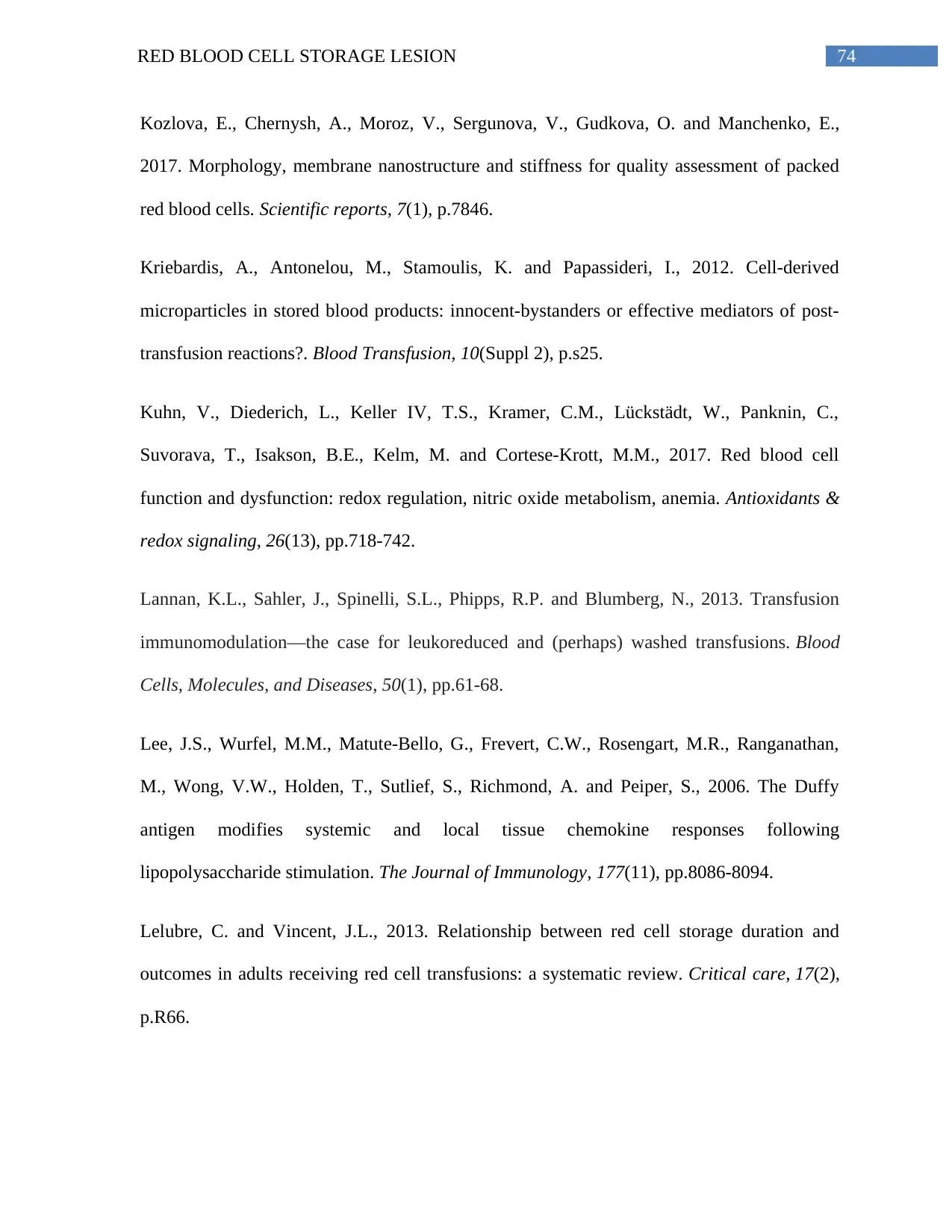
74RED BLOOD CELL STORAGE LESION
Kozlova, E., Chernysh, A., Moroz, V., Sergunova, V., Gudkova, O. and Manchenko, E.,
2017. Morphology, membrane nanostructure and stiffness for quality assessment of packed
red blood cells. Scientific reports, 7(1), p.7846.
Kriebardis, A., Antonelou, M., Stamoulis, K. and Papassideri, I., 2012. Cell-derived
microparticles in stored blood products: innocent-bystanders or effective mediators of post-
transfusion reactions?. Blood Transfusion, 10(Suppl 2), p.s25.
Kuhn, V., Diederich, L., Keller IV, T.S., Kramer, C.M., Lückstädt, W., Panknin, C.,
Suvorava, T., Isakson, B.E., Kelm, M. and Cortese-Krott, M.M., 2017. Red blood cell
function and dysfunction: redox regulation, nitric oxide metabolism, anemia. Antioxidants &
redox signaling, 26(13), pp.718-742.
Lannan, K.L., Sahler, J., Spinelli, S.L., Phipps, R.P. and Blumberg, N., 2013. Transfusion
immunomodulation—the case for leukoreduced and (perhaps) washed transfusions. Blood
Cells, Molecules, and Diseases, 50(1), pp.61-68.
Lee, J.S., Wurfel, M.M., Matute-Bello, G., Frevert, C.W., Rosengart, M.R., Ranganathan,
M., Wong, V.W., Holden, T., Sutlief, S., Richmond, A. and Peiper, S., 2006. The Duffy
antigen modifies systemic and local tissue chemokine responses following
lipopolysaccharide stimulation. The Journal of Immunology, 177(11), pp.8086-8094.
Lelubre, C. and Vincent, J.L., 2013. Relationship between red cell storage duration and
outcomes in adults receiving red cell transfusions: a systematic review. Critical care, 17(2),
p.R66.
Kozlova, E., Chernysh, A., Moroz, V., Sergunova, V., Gudkova, O. and Manchenko, E.,
2017. Morphology, membrane nanostructure and stiffness for quality assessment of packed
red blood cells. Scientific reports, 7(1), p.7846.
Kriebardis, A., Antonelou, M., Stamoulis, K. and Papassideri, I., 2012. Cell-derived
microparticles in stored blood products: innocent-bystanders or effective mediators of post-
transfusion reactions?. Blood Transfusion, 10(Suppl 2), p.s25.
Kuhn, V., Diederich, L., Keller IV, T.S., Kramer, C.M., Lückstädt, W., Panknin, C.,
Suvorava, T., Isakson, B.E., Kelm, M. and Cortese-Krott, M.M., 2017. Red blood cell
function and dysfunction: redox regulation, nitric oxide metabolism, anemia. Antioxidants &
redox signaling, 26(13), pp.718-742.
Lannan, K.L., Sahler, J., Spinelli, S.L., Phipps, R.P. and Blumberg, N., 2013. Transfusion
immunomodulation—the case for leukoreduced and (perhaps) washed transfusions. Blood
Cells, Molecules, and Diseases, 50(1), pp.61-68.
Lee, J.S., Wurfel, M.M., Matute-Bello, G., Frevert, C.W., Rosengart, M.R., Ranganathan,
M., Wong, V.W., Holden, T., Sutlief, S., Richmond, A. and Peiper, S., 2006. The Duffy
antigen modifies systemic and local tissue chemokine responses following
lipopolysaccharide stimulation. The Journal of Immunology, 177(11), pp.8086-8094.
Lelubre, C. and Vincent, J.L., 2013. Relationship between red cell storage duration and
outcomes in adults receiving red cell transfusions: a systematic review. Critical care, 17(2),
p.R66.
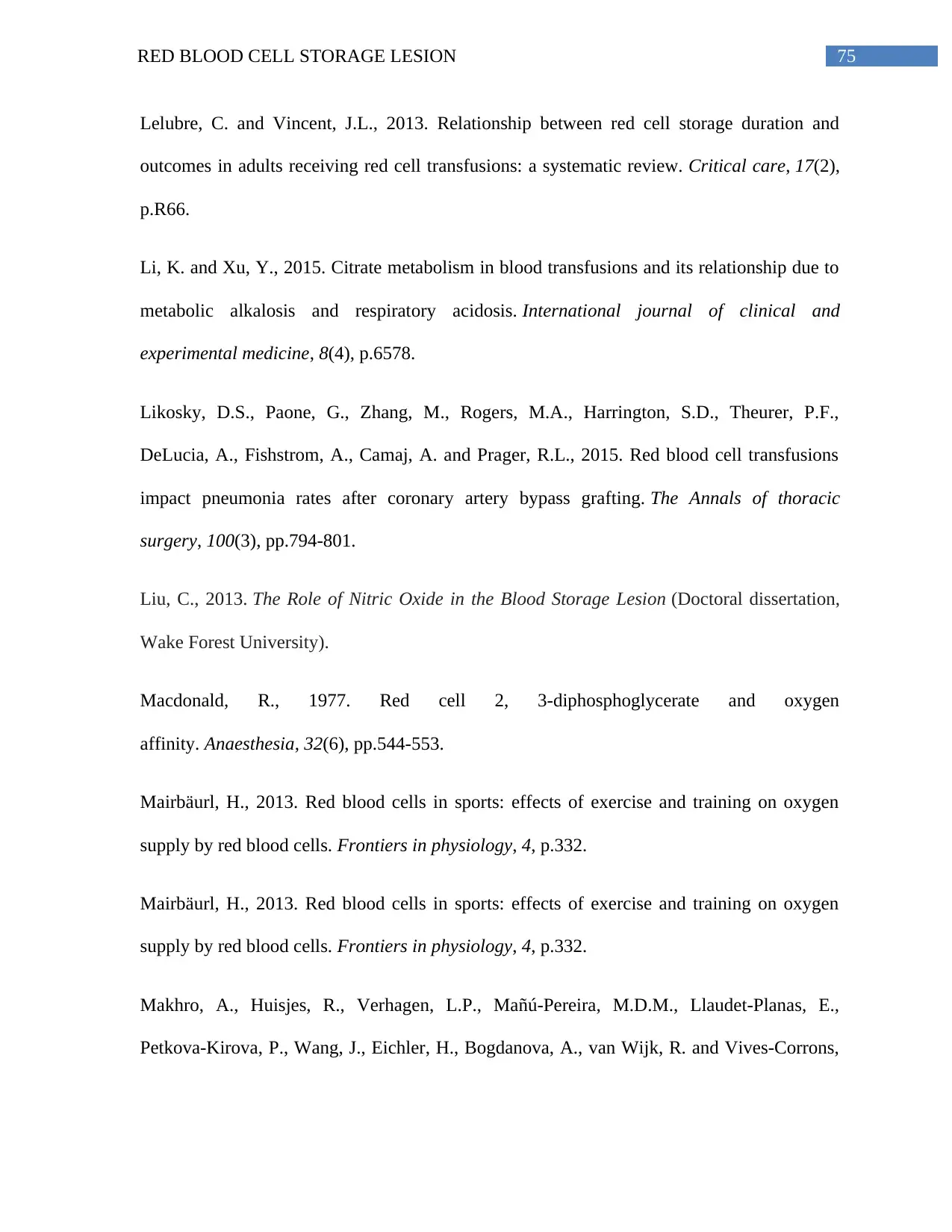
75RED BLOOD CELL STORAGE LESION
Lelubre, C. and Vincent, J.L., 2013. Relationship between red cell storage duration and
outcomes in adults receiving red cell transfusions: a systematic review. Critical care, 17(2),
p.R66.
Li, K. and Xu, Y., 2015. Citrate metabolism in blood transfusions and its relationship due to
metabolic alkalosis and respiratory acidosis. International journal of clinical and
experimental medicine, 8(4), p.6578.
Likosky, D.S., Paone, G., Zhang, M., Rogers, M.A., Harrington, S.D., Theurer, P.F.,
DeLucia, A., Fishstrom, A., Camaj, A. and Prager, R.L., 2015. Red blood cell transfusions
impact pneumonia rates after coronary artery bypass grafting. The Annals of thoracic
surgery, 100(3), pp.794-801.
Liu, C., 2013. The Role of Nitric Oxide in the Blood Storage Lesion (Doctoral dissertation,
Wake Forest University).
Macdonald, R., 1977. Red cell 2, 3‐diphosphoglycerate and oxygen
affinity. Anaesthesia, 32(6), pp.544-553.
Mairbäurl, H., 2013. Red blood cells in sports: effects of exercise and training on oxygen
supply by red blood cells. Frontiers in physiology, 4, p.332.
Mairbäurl, H., 2013. Red blood cells in sports: effects of exercise and training on oxygen
supply by red blood cells. Frontiers in physiology, 4, p.332.
Makhro, A., Huisjes, R., Verhagen, L.P., Mañú-Pereira, M.D.M., Llaudet-Planas, E.,
Petkova-Kirova, P., Wang, J., Eichler, H., Bogdanova, A., van Wijk, R. and Vives-Corrons,
Lelubre, C. and Vincent, J.L., 2013. Relationship between red cell storage duration and
outcomes in adults receiving red cell transfusions: a systematic review. Critical care, 17(2),
p.R66.
Li, K. and Xu, Y., 2015. Citrate metabolism in blood transfusions and its relationship due to
metabolic alkalosis and respiratory acidosis. International journal of clinical and
experimental medicine, 8(4), p.6578.
Likosky, D.S., Paone, G., Zhang, M., Rogers, M.A., Harrington, S.D., Theurer, P.F.,
DeLucia, A., Fishstrom, A., Camaj, A. and Prager, R.L., 2015. Red blood cell transfusions
impact pneumonia rates after coronary artery bypass grafting. The Annals of thoracic
surgery, 100(3), pp.794-801.
Liu, C., 2013. The Role of Nitric Oxide in the Blood Storage Lesion (Doctoral dissertation,
Wake Forest University).
Macdonald, R., 1977. Red cell 2, 3‐diphosphoglycerate and oxygen
affinity. Anaesthesia, 32(6), pp.544-553.
Mairbäurl, H., 2013. Red blood cells in sports: effects of exercise and training on oxygen
supply by red blood cells. Frontiers in physiology, 4, p.332.
Mairbäurl, H., 2013. Red blood cells in sports: effects of exercise and training on oxygen
supply by red blood cells. Frontiers in physiology, 4, p.332.
Makhro, A., Huisjes, R., Verhagen, L.P., Mañú-Pereira, M.D.M., Llaudet-Planas, E.,
Petkova-Kirova, P., Wang, J., Eichler, H., Bogdanova, A., van Wijk, R. and Vives-Corrons,
Secure Best Marks with AI Grader
Need help grading? Try our AI Grader for instant feedback on your assignments.
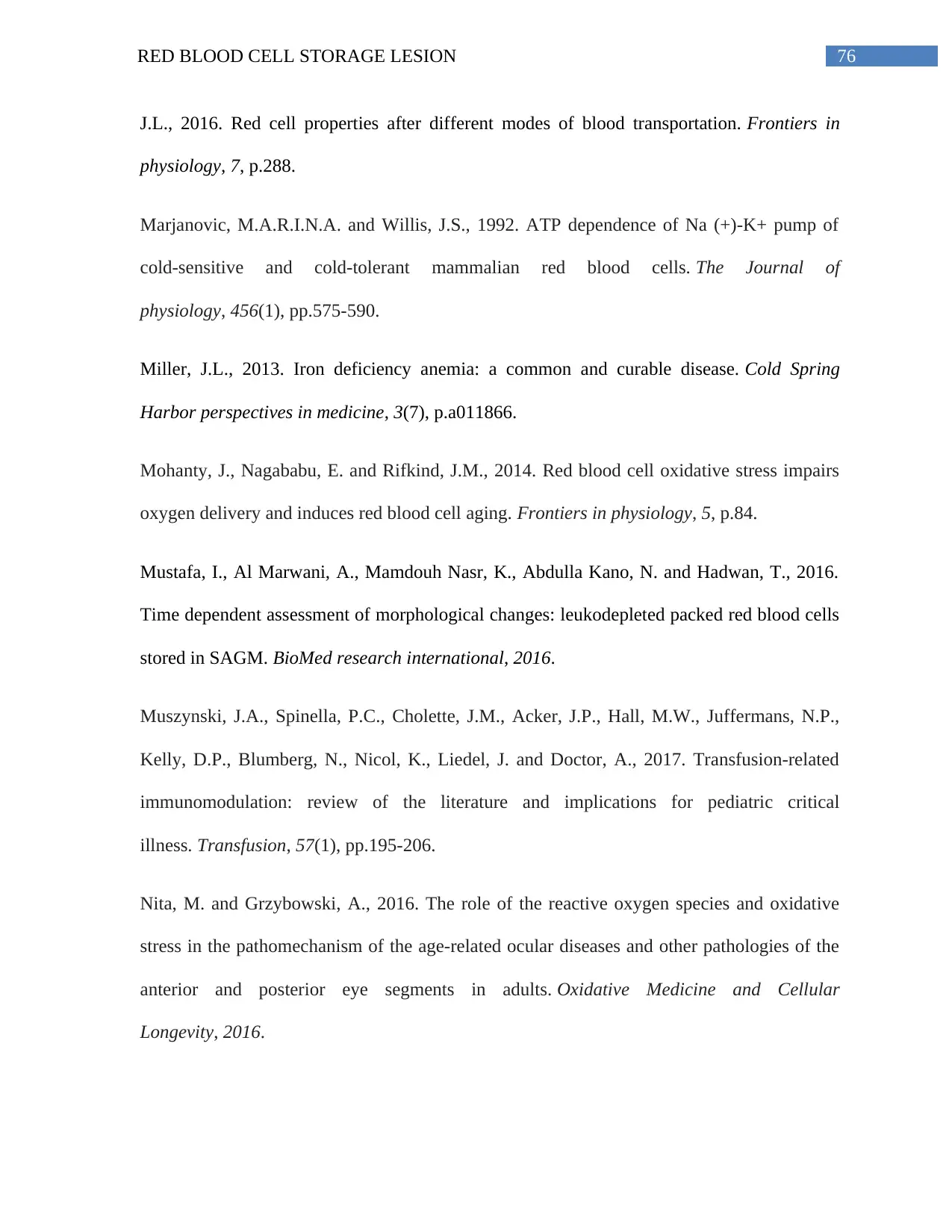
76RED BLOOD CELL STORAGE LESION
J.L., 2016. Red cell properties after different modes of blood transportation. Frontiers in
physiology, 7, p.288.
Marjanovic, M.A.R.I.N.A. and Willis, J.S., 1992. ATP dependence of Na (+)‐K+ pump of
cold‐sensitive and cold‐tolerant mammalian red blood cells. The Journal of
physiology, 456(1), pp.575-590.
Miller, J.L., 2013. Iron deficiency anemia: a common and curable disease. Cold Spring
Harbor perspectives in medicine, 3(7), p.a011866.
Mohanty, J., Nagababu, E. and Rifkind, J.M., 2014. Red blood cell oxidative stress impairs
oxygen delivery and induces red blood cell aging. Frontiers in physiology, 5, p.84.
Mustafa, I., Al Marwani, A., Mamdouh Nasr, K., Abdulla Kano, N. and Hadwan, T., 2016.
Time dependent assessment of morphological changes: leukodepleted packed red blood cells
stored in SAGM. BioMed research international, 2016.
Muszynski, J.A., Spinella, P.C., Cholette, J.M., Acker, J.P., Hall, M.W., Juffermans, N.P.,
Kelly, D.P., Blumberg, N., Nicol, K., Liedel, J. and Doctor, A., 2017. Transfusion‐related
immunomodulation: review of the literature and implications for pediatric critical
illness. Transfusion, 57(1), pp.195-206.
Nita, M. and Grzybowski, A., 2016. The role of the reactive oxygen species and oxidative
stress in the pathomechanism of the age-related ocular diseases and other pathologies of the
anterior and posterior eye segments in adults. Oxidative Medicine and Cellular
Longevity, 2016.
J.L., 2016. Red cell properties after different modes of blood transportation. Frontiers in
physiology, 7, p.288.
Marjanovic, M.A.R.I.N.A. and Willis, J.S., 1992. ATP dependence of Na (+)‐K+ pump of
cold‐sensitive and cold‐tolerant mammalian red blood cells. The Journal of
physiology, 456(1), pp.575-590.
Miller, J.L., 2013. Iron deficiency anemia: a common and curable disease. Cold Spring
Harbor perspectives in medicine, 3(7), p.a011866.
Mohanty, J., Nagababu, E. and Rifkind, J.M., 2014. Red blood cell oxidative stress impairs
oxygen delivery and induces red blood cell aging. Frontiers in physiology, 5, p.84.
Mustafa, I., Al Marwani, A., Mamdouh Nasr, K., Abdulla Kano, N. and Hadwan, T., 2016.
Time dependent assessment of morphological changes: leukodepleted packed red blood cells
stored in SAGM. BioMed research international, 2016.
Muszynski, J.A., Spinella, P.C., Cholette, J.M., Acker, J.P., Hall, M.W., Juffermans, N.P.,
Kelly, D.P., Blumberg, N., Nicol, K., Liedel, J. and Doctor, A., 2017. Transfusion‐related
immunomodulation: review of the literature and implications for pediatric critical
illness. Transfusion, 57(1), pp.195-206.
Nita, M. and Grzybowski, A., 2016. The role of the reactive oxygen species and oxidative
stress in the pathomechanism of the age-related ocular diseases and other pathologies of the
anterior and posterior eye segments in adults. Oxidative Medicine and Cellular
Longevity, 2016.
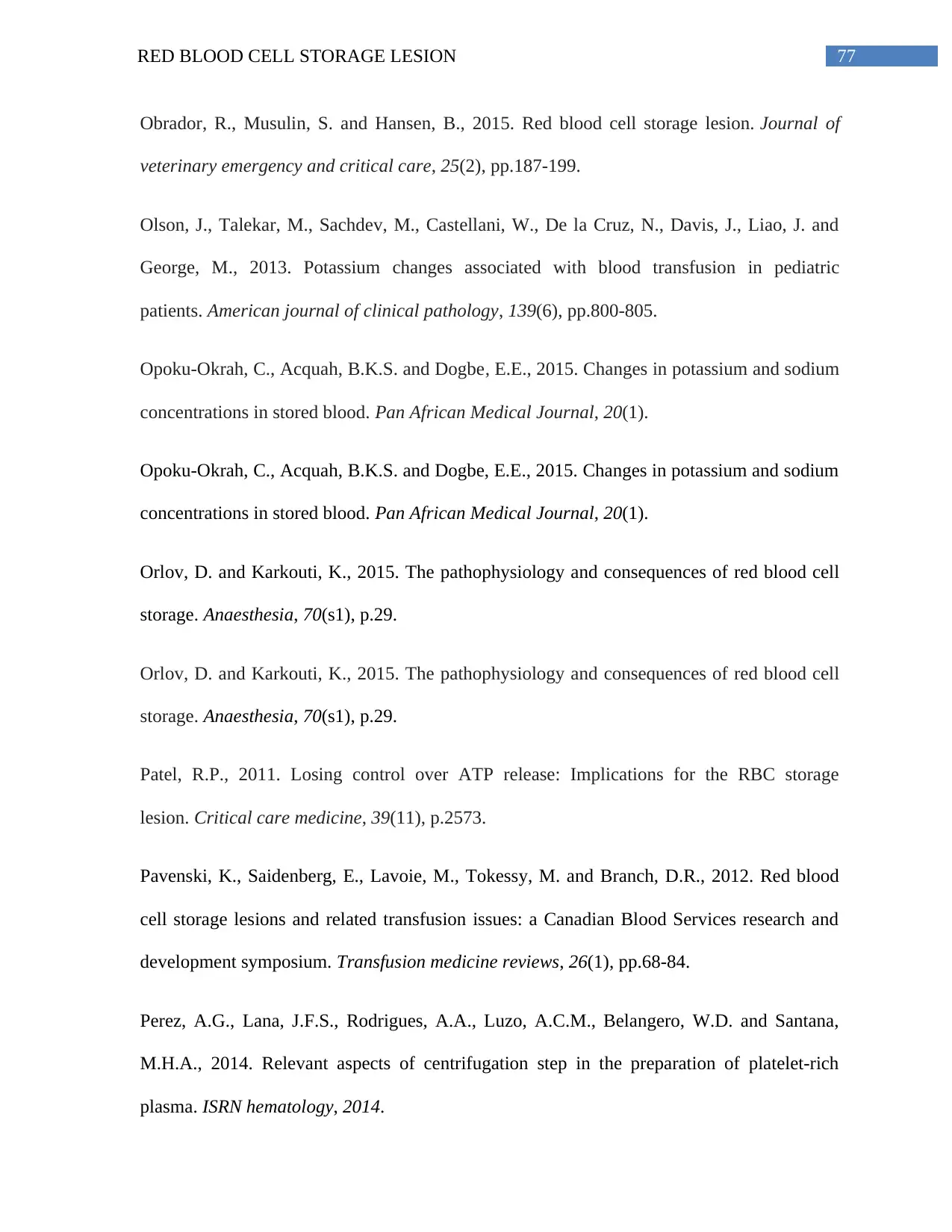
77RED BLOOD CELL STORAGE LESION
Obrador, R., Musulin, S. and Hansen, B., 2015. Red blood cell storage lesion. Journal of
veterinary emergency and critical care, 25(2), pp.187-199.
Olson, J., Talekar, M., Sachdev, M., Castellani, W., De la Cruz, N., Davis, J., Liao, J. and
George, M., 2013. Potassium changes associated with blood transfusion in pediatric
patients. American journal of clinical pathology, 139(6), pp.800-805.
Opoku-Okrah, C., Acquah, B.K.S. and Dogbe, E.E., 2015. Changes in potassium and sodium
concentrations in stored blood. Pan African Medical Journal, 20(1).
Opoku-Okrah, C., Acquah, B.K.S. and Dogbe, E.E., 2015. Changes in potassium and sodium
concentrations in stored blood. Pan African Medical Journal, 20(1).
Orlov, D. and Karkouti, K., 2015. The pathophysiology and consequences of red blood cell
storage. Anaesthesia, 70(s1), p.29.
Orlov, D. and Karkouti, K., 2015. The pathophysiology and consequences of red blood cell
storage. Anaesthesia, 70(s1), p.29.
Patel, R.P., 2011. Losing control over ATP release: Implications for the RBC storage
lesion. Critical care medicine, 39(11), p.2573.
Pavenski, K., Saidenberg, E., Lavoie, M., Tokessy, M. and Branch, D.R., 2012. Red blood
cell storage lesions and related transfusion issues: a Canadian Blood Services research and
development symposium. Transfusion medicine reviews, 26(1), pp.68-84.
Perez, A.G., Lana, J.F.S., Rodrigues, A.A., Luzo, A.C.M., Belangero, W.D. and Santana,
M.H.A., 2014. Relevant aspects of centrifugation step in the preparation of platelet-rich
plasma. ISRN hematology, 2014.
Obrador, R., Musulin, S. and Hansen, B., 2015. Red blood cell storage lesion. Journal of
veterinary emergency and critical care, 25(2), pp.187-199.
Olson, J., Talekar, M., Sachdev, M., Castellani, W., De la Cruz, N., Davis, J., Liao, J. and
George, M., 2013. Potassium changes associated with blood transfusion in pediatric
patients. American journal of clinical pathology, 139(6), pp.800-805.
Opoku-Okrah, C., Acquah, B.K.S. and Dogbe, E.E., 2015. Changes in potassium and sodium
concentrations in stored blood. Pan African Medical Journal, 20(1).
Opoku-Okrah, C., Acquah, B.K.S. and Dogbe, E.E., 2015. Changes in potassium and sodium
concentrations in stored blood. Pan African Medical Journal, 20(1).
Orlov, D. and Karkouti, K., 2015. The pathophysiology and consequences of red blood cell
storage. Anaesthesia, 70(s1), p.29.
Orlov, D. and Karkouti, K., 2015. The pathophysiology and consequences of red blood cell
storage. Anaesthesia, 70(s1), p.29.
Patel, R.P., 2011. Losing control over ATP release: Implications for the RBC storage
lesion. Critical care medicine, 39(11), p.2573.
Pavenski, K., Saidenberg, E., Lavoie, M., Tokessy, M. and Branch, D.R., 2012. Red blood
cell storage lesions and related transfusion issues: a Canadian Blood Services research and
development symposium. Transfusion medicine reviews, 26(1), pp.68-84.
Perez, A.G., Lana, J.F.S., Rodrigues, A.A., Luzo, A.C.M., Belangero, W.D. and Santana,
M.H.A., 2014. Relevant aspects of centrifugation step in the preparation of platelet-rich
plasma. ISRN hematology, 2014.
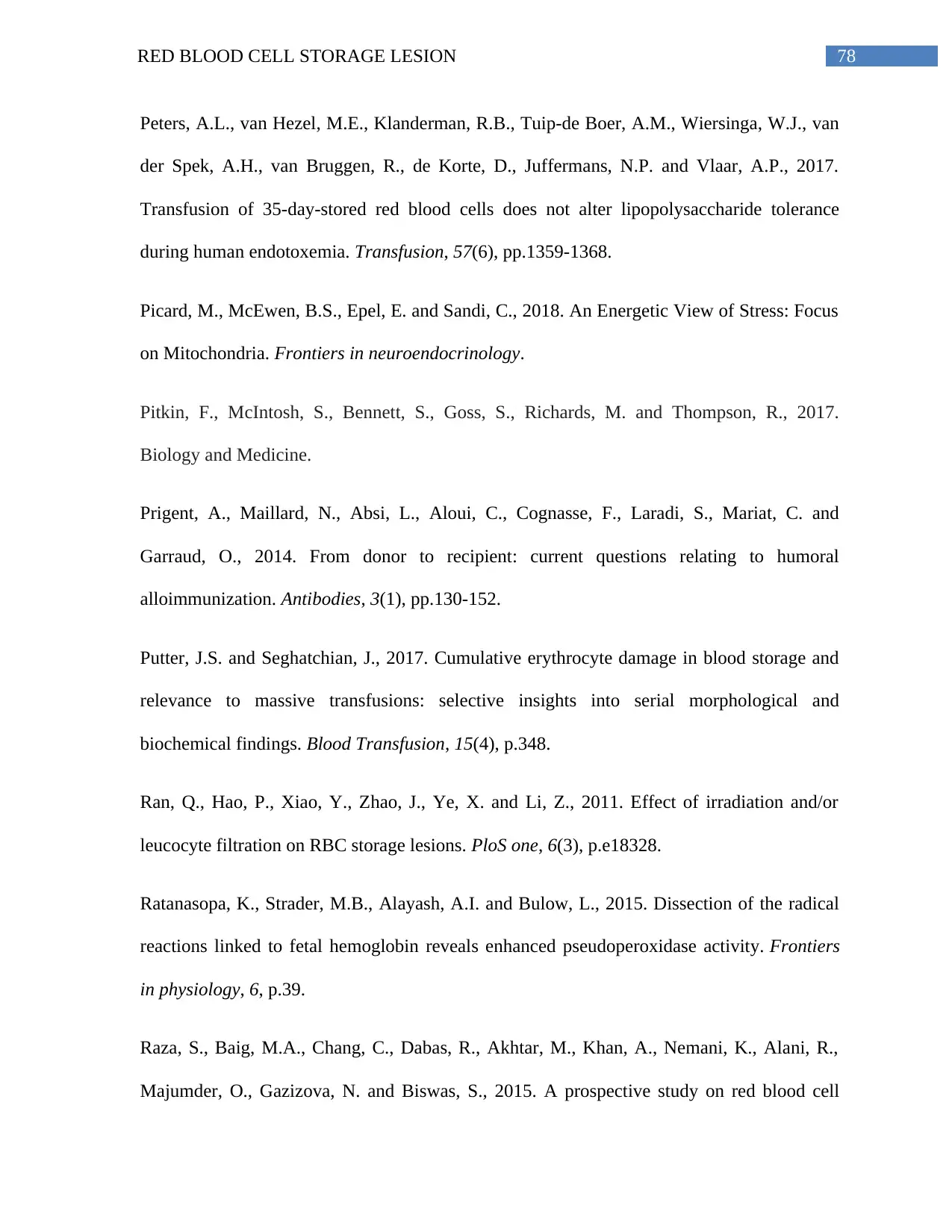
78RED BLOOD CELL STORAGE LESION
Peters, A.L., van Hezel, M.E., Klanderman, R.B., Tuip‐de Boer, A.M., Wiersinga, W.J., van
der Spek, A.H., van Bruggen, R., de Korte, D., Juffermans, N.P. and Vlaar, A.P., 2017.
Transfusion of 35‐day‐stored red blood cells does not alter lipopolysaccharide tolerance
during human endotoxemia. Transfusion, 57(6), pp.1359-1368.
Picard, M., McEwen, B.S., Epel, E. and Sandi, C., 2018. An Energetic View of Stress: Focus
on Mitochondria. Frontiers in neuroendocrinology.
Pitkin, F., McIntosh, S., Bennett, S., Goss, S., Richards, M. and Thompson, R., 2017.
Biology and Medicine.
Prigent, A., Maillard, N., Absi, L., Aloui, C., Cognasse, F., Laradi, S., Mariat, C. and
Garraud, O., 2014. From donor to recipient: current questions relating to humoral
alloimmunization. Antibodies, 3(1), pp.130-152.
Putter, J.S. and Seghatchian, J., 2017. Cumulative erythrocyte damage in blood storage and
relevance to massive transfusions: selective insights into serial morphological and
biochemical findings. Blood Transfusion, 15(4), p.348.
Ran, Q., Hao, P., Xiao, Y., Zhao, J., Ye, X. and Li, Z., 2011. Effect of irradiation and/or
leucocyte filtration on RBC storage lesions. PloS one, 6(3), p.e18328.
Ratanasopa, K., Strader, M.B., Alayash, A.I. and Bulow, L., 2015. Dissection of the radical
reactions linked to fetal hemoglobin reveals enhanced pseudoperoxidase activity. Frontiers
in physiology, 6, p.39.
Raza, S., Baig, M.A., Chang, C., Dabas, R., Akhtar, M., Khan, A., Nemani, K., Alani, R.,
Majumder, O., Gazizova, N. and Biswas, S., 2015. A prospective study on red blood cell
Peters, A.L., van Hezel, M.E., Klanderman, R.B., Tuip‐de Boer, A.M., Wiersinga, W.J., van
der Spek, A.H., van Bruggen, R., de Korte, D., Juffermans, N.P. and Vlaar, A.P., 2017.
Transfusion of 35‐day‐stored red blood cells does not alter lipopolysaccharide tolerance
during human endotoxemia. Transfusion, 57(6), pp.1359-1368.
Picard, M., McEwen, B.S., Epel, E. and Sandi, C., 2018. An Energetic View of Stress: Focus
on Mitochondria. Frontiers in neuroendocrinology.
Pitkin, F., McIntosh, S., Bennett, S., Goss, S., Richards, M. and Thompson, R., 2017.
Biology and Medicine.
Prigent, A., Maillard, N., Absi, L., Aloui, C., Cognasse, F., Laradi, S., Mariat, C. and
Garraud, O., 2014. From donor to recipient: current questions relating to humoral
alloimmunization. Antibodies, 3(1), pp.130-152.
Putter, J.S. and Seghatchian, J., 2017. Cumulative erythrocyte damage in blood storage and
relevance to massive transfusions: selective insights into serial morphological and
biochemical findings. Blood Transfusion, 15(4), p.348.
Ran, Q., Hao, P., Xiao, Y., Zhao, J., Ye, X. and Li, Z., 2011. Effect of irradiation and/or
leucocyte filtration on RBC storage lesions. PloS one, 6(3), p.e18328.
Ratanasopa, K., Strader, M.B., Alayash, A.I. and Bulow, L., 2015. Dissection of the radical
reactions linked to fetal hemoglobin reveals enhanced pseudoperoxidase activity. Frontiers
in physiology, 6, p.39.
Raza, S., Baig, M.A., Chang, C., Dabas, R., Akhtar, M., Khan, A., Nemani, K., Alani, R.,
Majumder, O., Gazizova, N. and Biswas, S., 2015. A prospective study on red blood cell
Paraphrase This Document
Need a fresh take? Get an instant paraphrase of this document with our AI Paraphraser
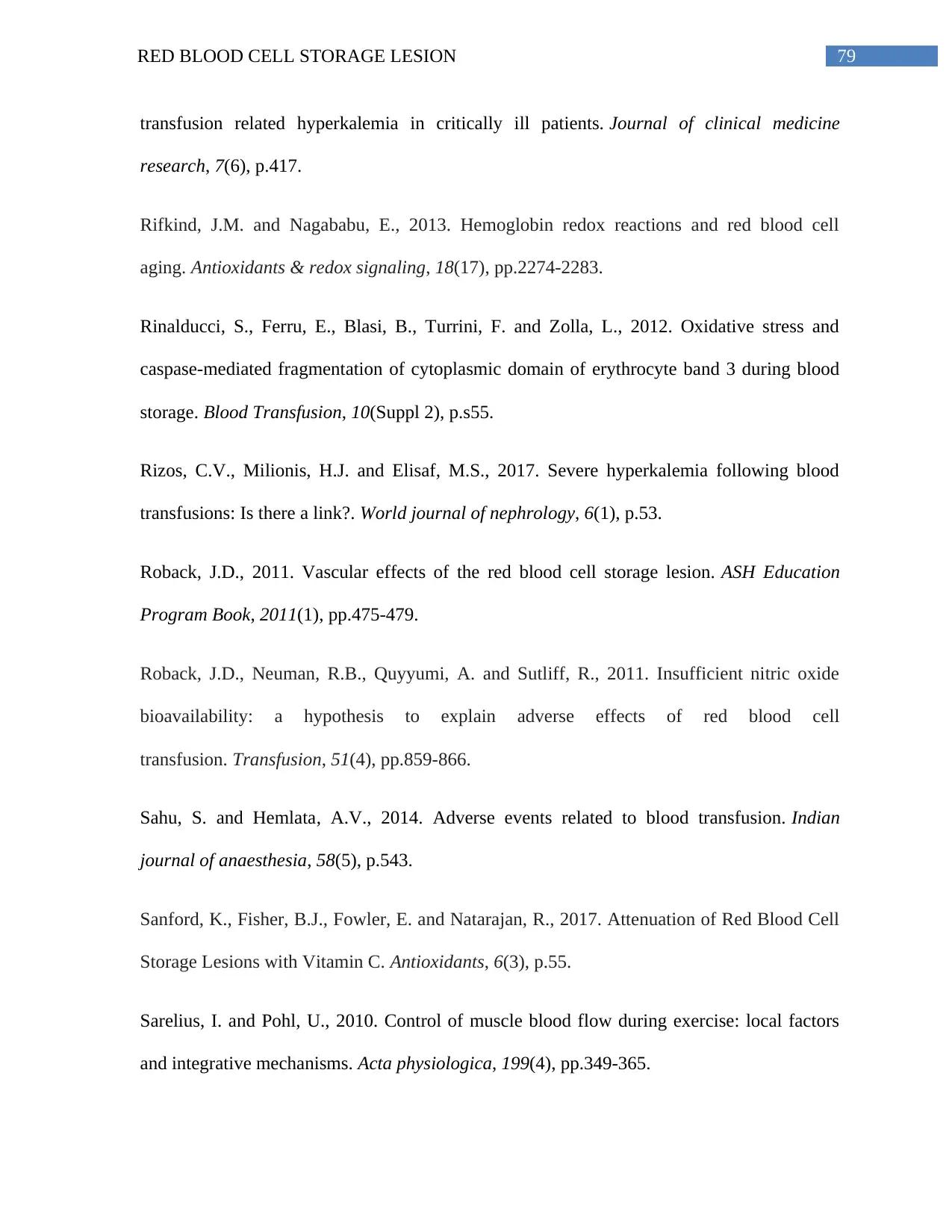
79RED BLOOD CELL STORAGE LESION
transfusion related hyperkalemia in critically ill patients. Journal of clinical medicine
research, 7(6), p.417.
Rifkind, J.M. and Nagababu, E., 2013. Hemoglobin redox reactions and red blood cell
aging. Antioxidants & redox signaling, 18(17), pp.2274-2283.
Rinalducci, S., Ferru, E., Blasi, B., Turrini, F. and Zolla, L., 2012. Oxidative stress and
caspase-mediated fragmentation of cytoplasmic domain of erythrocyte band 3 during blood
storage. Blood Transfusion, 10(Suppl 2), p.s55.
Rizos, C.V., Milionis, H.J. and Elisaf, M.S., 2017. Severe hyperkalemia following blood
transfusions: Is there a link?. World journal of nephrology, 6(1), p.53.
Roback, J.D., 2011. Vascular effects of the red blood cell storage lesion. ASH Education
Program Book, 2011(1), pp.475-479.
Roback, J.D., Neuman, R.B., Quyyumi, A. and Sutliff, R., 2011. Insufficient nitric oxide
bioavailability: a hypothesis to explain adverse effects of red blood cell
transfusion. Transfusion, 51(4), pp.859-866.
Sahu, S. and Hemlata, A.V., 2014. Adverse events related to blood transfusion. Indian
journal of anaesthesia, 58(5), p.543.
Sanford, K., Fisher, B.J., Fowler, E. and Natarajan, R., 2017. Attenuation of Red Blood Cell
Storage Lesions with Vitamin C. Antioxidants, 6(3), p.55.
Sarelius, I. and Pohl, U., 2010. Control of muscle blood flow during exercise: local factors
and integrative mechanisms. Acta physiologica, 199(4), pp.349-365.
transfusion related hyperkalemia in critically ill patients. Journal of clinical medicine
research, 7(6), p.417.
Rifkind, J.M. and Nagababu, E., 2013. Hemoglobin redox reactions and red blood cell
aging. Antioxidants & redox signaling, 18(17), pp.2274-2283.
Rinalducci, S., Ferru, E., Blasi, B., Turrini, F. and Zolla, L., 2012. Oxidative stress and
caspase-mediated fragmentation of cytoplasmic domain of erythrocyte band 3 during blood
storage. Blood Transfusion, 10(Suppl 2), p.s55.
Rizos, C.V., Milionis, H.J. and Elisaf, M.S., 2017. Severe hyperkalemia following blood
transfusions: Is there a link?. World journal of nephrology, 6(1), p.53.
Roback, J.D., 2011. Vascular effects of the red blood cell storage lesion. ASH Education
Program Book, 2011(1), pp.475-479.
Roback, J.D., Neuman, R.B., Quyyumi, A. and Sutliff, R., 2011. Insufficient nitric oxide
bioavailability: a hypothesis to explain adverse effects of red blood cell
transfusion. Transfusion, 51(4), pp.859-866.
Sahu, S. and Hemlata, A.V., 2014. Adverse events related to blood transfusion. Indian
journal of anaesthesia, 58(5), p.543.
Sanford, K., Fisher, B.J., Fowler, E. and Natarajan, R., 2017. Attenuation of Red Blood Cell
Storage Lesions with Vitamin C. Antioxidants, 6(3), p.55.
Sarelius, I. and Pohl, U., 2010. Control of muscle blood flow during exercise: local factors
and integrative mechanisms. Acta physiologica, 199(4), pp.349-365.
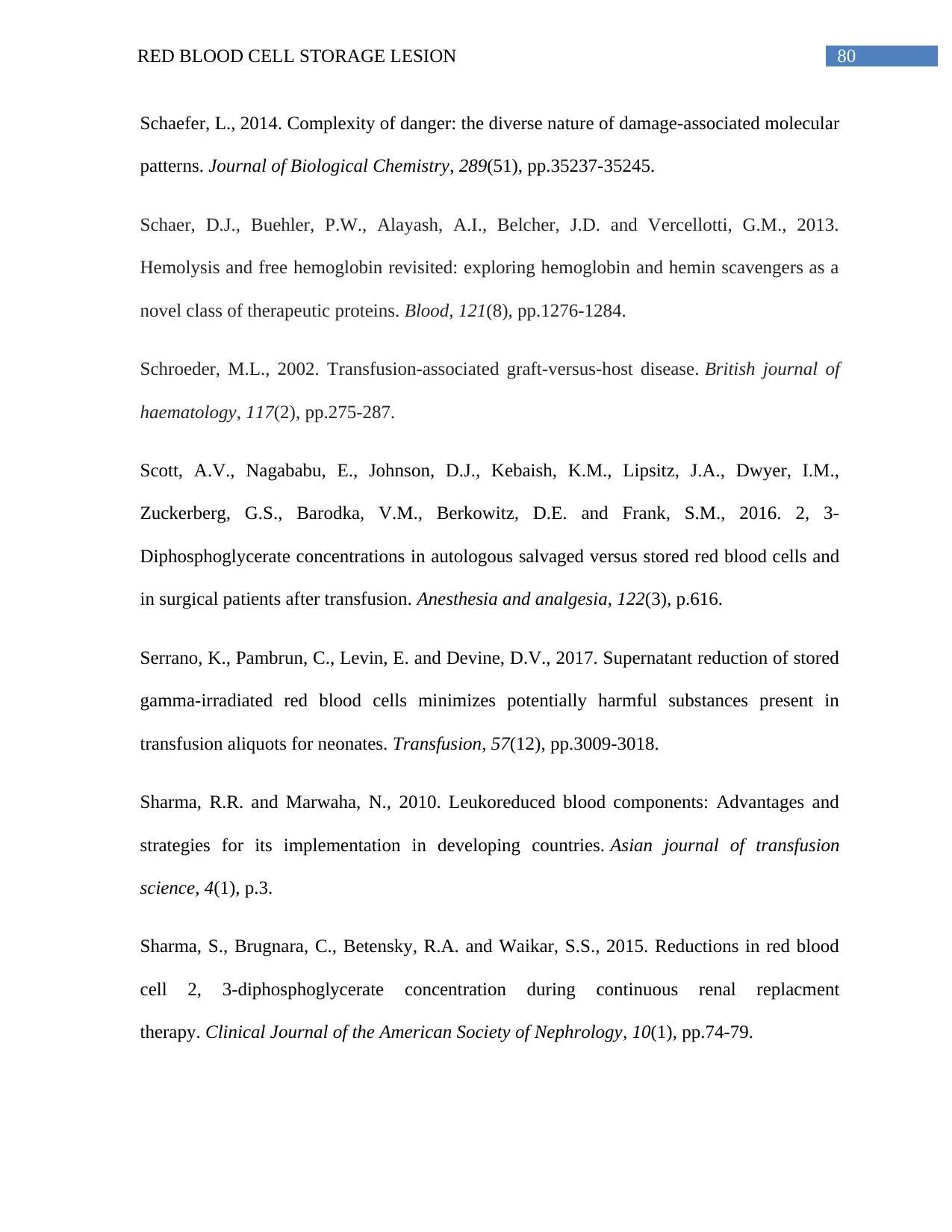
80RED BLOOD CELL STORAGE LESION
Schaefer, L., 2014. Complexity of danger: the diverse nature of damage-associated molecular
patterns. Journal of Biological Chemistry, 289(51), pp.35237-35245.
Schaer, D.J., Buehler, P.W., Alayash, A.I., Belcher, J.D. and Vercellotti, G.M., 2013.
Hemolysis and free hemoglobin revisited: exploring hemoglobin and hemin scavengers as a
novel class of therapeutic proteins. Blood, 121(8), pp.1276-1284.
Schroeder, M.L., 2002. Transfusion‐associated graft‐versus‐host disease. British journal of
haematology, 117(2), pp.275-287.
Scott, A.V., Nagababu, E., Johnson, D.J., Kebaish, K.M., Lipsitz, J.A., Dwyer, I.M.,
Zuckerberg, G.S., Barodka, V.M., Berkowitz, D.E. and Frank, S.M., 2016. 2, 3-
Diphosphoglycerate concentrations in autologous salvaged versus stored red blood cells and
in surgical patients after transfusion. Anesthesia and analgesia, 122(3), p.616.
Serrano, K., Pambrun, C., Levin, E. and Devine, D.V., 2017. Supernatant reduction of stored
gamma‐irradiated red blood cells minimizes potentially harmful substances present in
transfusion aliquots for neonates. Transfusion, 57(12), pp.3009-3018.
Sharma, R.R. and Marwaha, N., 2010. Leukoreduced blood components: Advantages and
strategies for its implementation in developing countries. Asian journal of transfusion
science, 4(1), p.3.
Sharma, S., Brugnara, C., Betensky, R.A. and Waikar, S.S., 2015. Reductions in red blood
cell 2, 3-diphosphoglycerate concentration during continuous renal replacment
therapy. Clinical Journal of the American Society of Nephrology, 10(1), pp.74-79.
Schaefer, L., 2014. Complexity of danger: the diverse nature of damage-associated molecular
patterns. Journal of Biological Chemistry, 289(51), pp.35237-35245.
Schaer, D.J., Buehler, P.W., Alayash, A.I., Belcher, J.D. and Vercellotti, G.M., 2013.
Hemolysis and free hemoglobin revisited: exploring hemoglobin and hemin scavengers as a
novel class of therapeutic proteins. Blood, 121(8), pp.1276-1284.
Schroeder, M.L., 2002. Transfusion‐associated graft‐versus‐host disease. British journal of
haematology, 117(2), pp.275-287.
Scott, A.V., Nagababu, E., Johnson, D.J., Kebaish, K.M., Lipsitz, J.A., Dwyer, I.M.,
Zuckerberg, G.S., Barodka, V.M., Berkowitz, D.E. and Frank, S.M., 2016. 2, 3-
Diphosphoglycerate concentrations in autologous salvaged versus stored red blood cells and
in surgical patients after transfusion. Anesthesia and analgesia, 122(3), p.616.
Serrano, K., Pambrun, C., Levin, E. and Devine, D.V., 2017. Supernatant reduction of stored
gamma‐irradiated red blood cells minimizes potentially harmful substances present in
transfusion aliquots for neonates. Transfusion, 57(12), pp.3009-3018.
Sharma, R.R. and Marwaha, N., 2010. Leukoreduced blood components: Advantages and
strategies for its implementation in developing countries. Asian journal of transfusion
science, 4(1), p.3.
Sharma, S., Brugnara, C., Betensky, R.A. and Waikar, S.S., 2015. Reductions in red blood
cell 2, 3-diphosphoglycerate concentration during continuous renal replacment
therapy. Clinical Journal of the American Society of Nephrology, 10(1), pp.74-79.
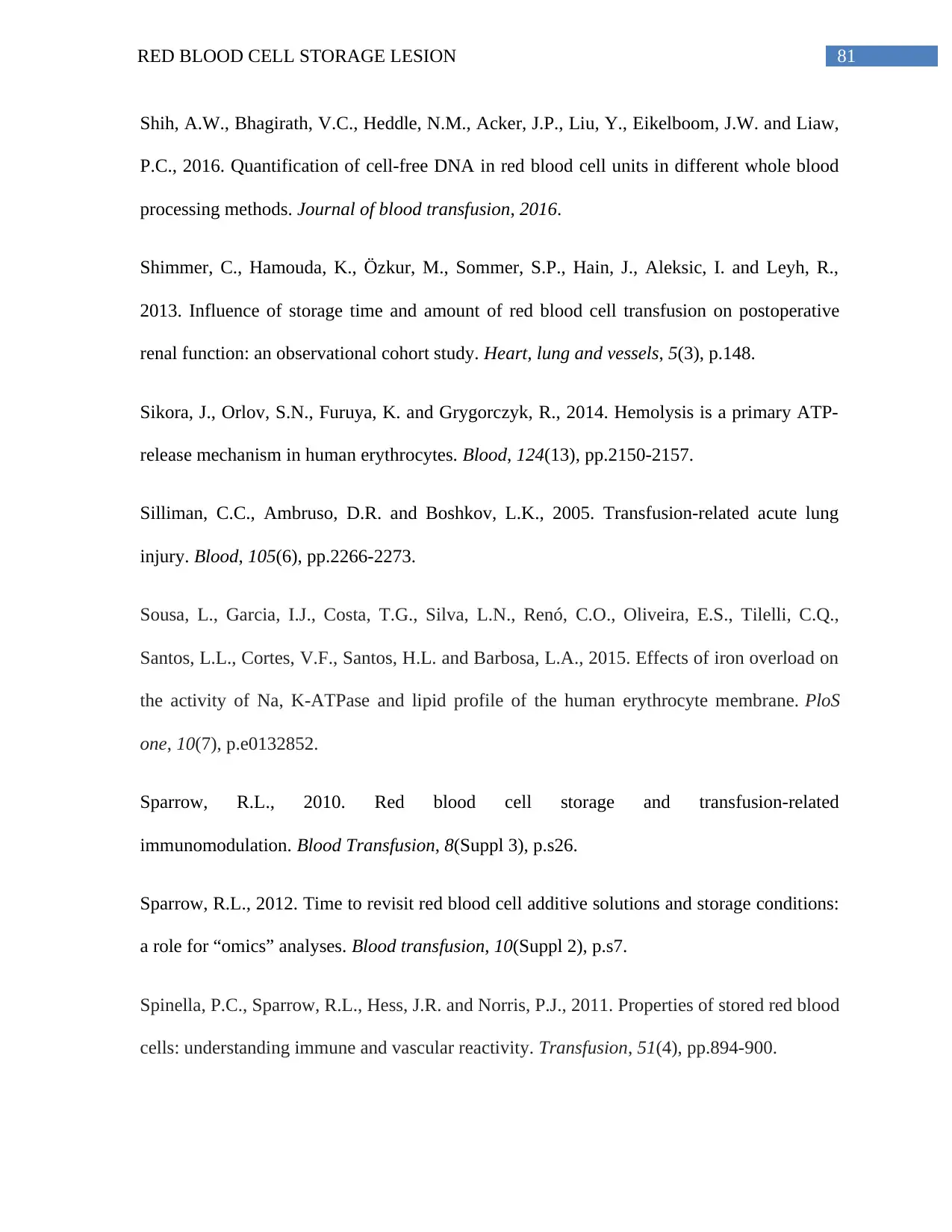
81RED BLOOD CELL STORAGE LESION
Shih, A.W., Bhagirath, V.C., Heddle, N.M., Acker, J.P., Liu, Y., Eikelboom, J.W. and Liaw,
P.C., 2016. Quantification of cell-free DNA in red blood cell units in different whole blood
processing methods. Journal of blood transfusion, 2016.
Shimmer, C., Hamouda, K., Özkur, M., Sommer, S.P., Hain, J., Aleksic, I. and Leyh, R.,
2013. Influence of storage time and amount of red blood cell transfusion on postoperative
renal function: an observational cohort study. Heart, lung and vessels, 5(3), p.148.
Sikora, J., Orlov, S.N., Furuya, K. and Grygorczyk, R., 2014. Hemolysis is a primary ATP-
release mechanism in human erythrocytes. Blood, 124(13), pp.2150-2157.
Silliman, C.C., Ambruso, D.R. and Boshkov, L.K., 2005. Transfusion-related acute lung
injury. Blood, 105(6), pp.2266-2273.
Sousa, L., Garcia, I.J., Costa, T.G., Silva, L.N., Renó, C.O., Oliveira, E.S., Tilelli, C.Q.,
Santos, L.L., Cortes, V.F., Santos, H.L. and Barbosa, L.A., 2015. Effects of iron overload on
the activity of Na, K-ATPase and lipid profile of the human erythrocyte membrane. PloS
one, 10(7), p.e0132852.
Sparrow, R.L., 2010. Red blood cell storage and transfusion-related
immunomodulation. Blood Transfusion, 8(Suppl 3), p.s26.
Sparrow, R.L., 2012. Time to revisit red blood cell additive solutions and storage conditions:
a role for “omics” analyses. Blood transfusion, 10(Suppl 2), p.s7.
Spinella, P.C., Sparrow, R.L., Hess, J.R. and Norris, P.J., 2011. Properties of stored red blood
cells: understanding immune and vascular reactivity. Transfusion, 51(4), pp.894-900.
Shih, A.W., Bhagirath, V.C., Heddle, N.M., Acker, J.P., Liu, Y., Eikelboom, J.W. and Liaw,
P.C., 2016. Quantification of cell-free DNA in red blood cell units in different whole blood
processing methods. Journal of blood transfusion, 2016.
Shimmer, C., Hamouda, K., Özkur, M., Sommer, S.P., Hain, J., Aleksic, I. and Leyh, R.,
2013. Influence of storage time and amount of red blood cell transfusion on postoperative
renal function: an observational cohort study. Heart, lung and vessels, 5(3), p.148.
Sikora, J., Orlov, S.N., Furuya, K. and Grygorczyk, R., 2014. Hemolysis is a primary ATP-
release mechanism in human erythrocytes. Blood, 124(13), pp.2150-2157.
Silliman, C.C., Ambruso, D.R. and Boshkov, L.K., 2005. Transfusion-related acute lung
injury. Blood, 105(6), pp.2266-2273.
Sousa, L., Garcia, I.J., Costa, T.G., Silva, L.N., Renó, C.O., Oliveira, E.S., Tilelli, C.Q.,
Santos, L.L., Cortes, V.F., Santos, H.L. and Barbosa, L.A., 2015. Effects of iron overload on
the activity of Na, K-ATPase and lipid profile of the human erythrocyte membrane. PloS
one, 10(7), p.e0132852.
Sparrow, R.L., 2010. Red blood cell storage and transfusion-related
immunomodulation. Blood Transfusion, 8(Suppl 3), p.s26.
Sparrow, R.L., 2012. Time to revisit red blood cell additive solutions and storage conditions:
a role for “omics” analyses. Blood transfusion, 10(Suppl 2), p.s7.
Spinella, P.C., Sparrow, R.L., Hess, J.R. and Norris, P.J., 2011. Properties of stored red blood
cells: understanding immune and vascular reactivity. Transfusion, 51(4), pp.894-900.
Secure Best Marks with AI Grader
Need help grading? Try our AI Grader for instant feedback on your assignments.
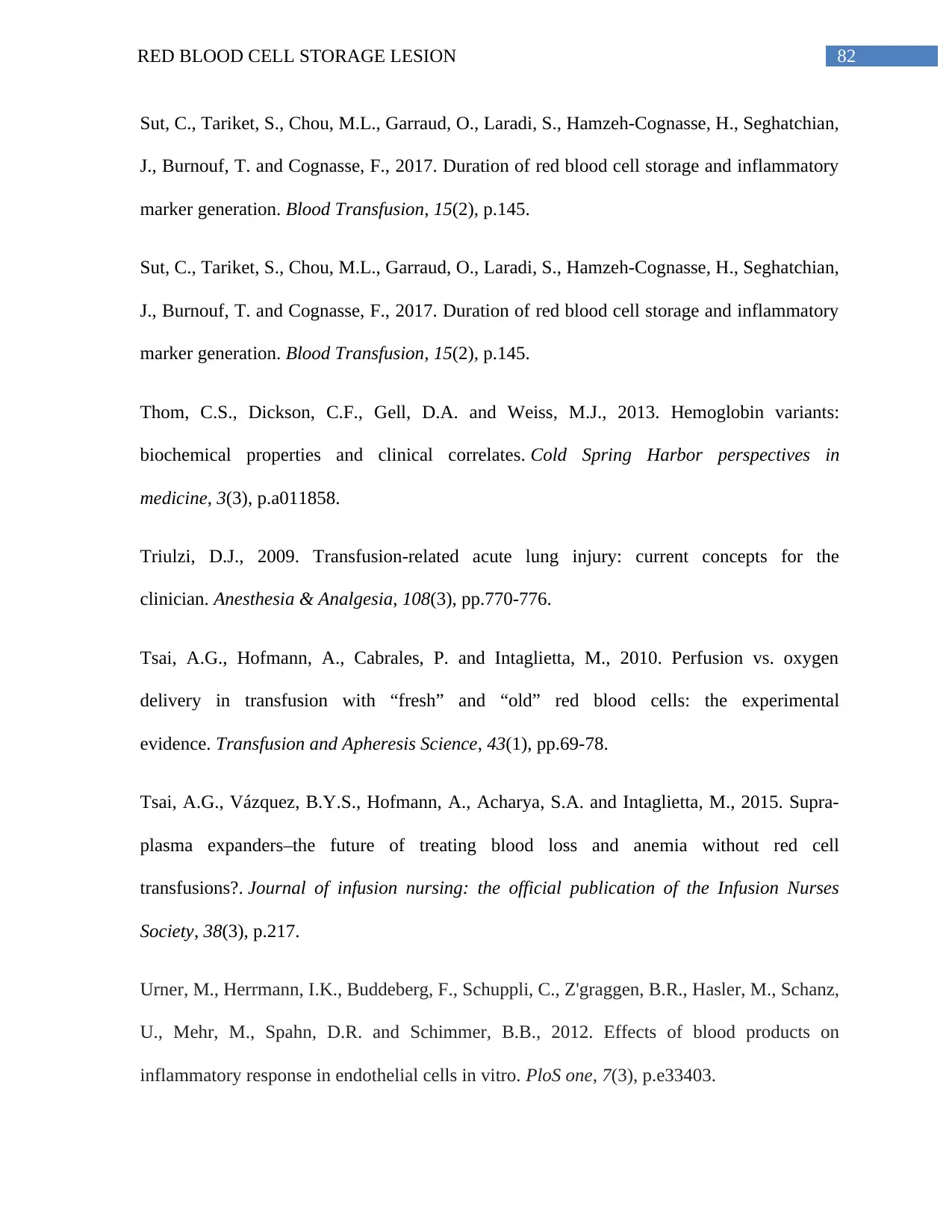
82RED BLOOD CELL STORAGE LESION
Sut, C., Tariket, S., Chou, M.L., Garraud, O., Laradi, S., Hamzeh-Cognasse, H., Seghatchian,
J., Burnouf, T. and Cognasse, F., 2017. Duration of red blood cell storage and inflammatory
marker generation. Blood Transfusion, 15(2), p.145.
Sut, C., Tariket, S., Chou, M.L., Garraud, O., Laradi, S., Hamzeh-Cognasse, H., Seghatchian,
J., Burnouf, T. and Cognasse, F., 2017. Duration of red blood cell storage and inflammatory
marker generation. Blood Transfusion, 15(2), p.145.
Thom, C.S., Dickson, C.F., Gell, D.A. and Weiss, M.J., 2013. Hemoglobin variants:
biochemical properties and clinical correlates. Cold Spring Harbor perspectives in
medicine, 3(3), p.a011858.
Triulzi, D.J., 2009. Transfusion-related acute lung injury: current concepts for the
clinician. Anesthesia & Analgesia, 108(3), pp.770-776.
Tsai, A.G., Hofmann, A., Cabrales, P. and Intaglietta, M., 2010. Perfusion vs. oxygen
delivery in transfusion with “fresh” and “old” red blood cells: the experimental
evidence. Transfusion and Apheresis Science, 43(1), pp.69-78.
Tsai, A.G., Vázquez, B.Y.S., Hofmann, A., Acharya, S.A. and Intaglietta, M., 2015. Supra-
plasma expanders–the future of treating blood loss and anemia without red cell
transfusions?. Journal of infusion nursing: the official publication of the Infusion Nurses
Society, 38(3), p.217.
Urner, M., Herrmann, I.K., Buddeberg, F., Schuppli, C., Z'graggen, B.R., Hasler, M., Schanz,
U., Mehr, M., Spahn, D.R. and Schimmer, B.B., 2012. Effects of blood products on
inflammatory response in endothelial cells in vitro. PloS one, 7(3), p.e33403.
Sut, C., Tariket, S., Chou, M.L., Garraud, O., Laradi, S., Hamzeh-Cognasse, H., Seghatchian,
J., Burnouf, T. and Cognasse, F., 2017. Duration of red blood cell storage and inflammatory
marker generation. Blood Transfusion, 15(2), p.145.
Sut, C., Tariket, S., Chou, M.L., Garraud, O., Laradi, S., Hamzeh-Cognasse, H., Seghatchian,
J., Burnouf, T. and Cognasse, F., 2017. Duration of red blood cell storage and inflammatory
marker generation. Blood Transfusion, 15(2), p.145.
Thom, C.S., Dickson, C.F., Gell, D.A. and Weiss, M.J., 2013. Hemoglobin variants:
biochemical properties and clinical correlates. Cold Spring Harbor perspectives in
medicine, 3(3), p.a011858.
Triulzi, D.J., 2009. Transfusion-related acute lung injury: current concepts for the
clinician. Anesthesia & Analgesia, 108(3), pp.770-776.
Tsai, A.G., Hofmann, A., Cabrales, P. and Intaglietta, M., 2010. Perfusion vs. oxygen
delivery in transfusion with “fresh” and “old” red blood cells: the experimental
evidence. Transfusion and Apheresis Science, 43(1), pp.69-78.
Tsai, A.G., Vázquez, B.Y.S., Hofmann, A., Acharya, S.A. and Intaglietta, M., 2015. Supra-
plasma expanders–the future of treating blood loss and anemia without red cell
transfusions?. Journal of infusion nursing: the official publication of the Infusion Nurses
Society, 38(3), p.217.
Urner, M., Herrmann, I.K., Buddeberg, F., Schuppli, C., Z'graggen, B.R., Hasler, M., Schanz,
U., Mehr, M., Spahn, D.R. and Schimmer, B.B., 2012. Effects of blood products on
inflammatory response in endothelial cells in vitro. PloS one, 7(3), p.e33403.
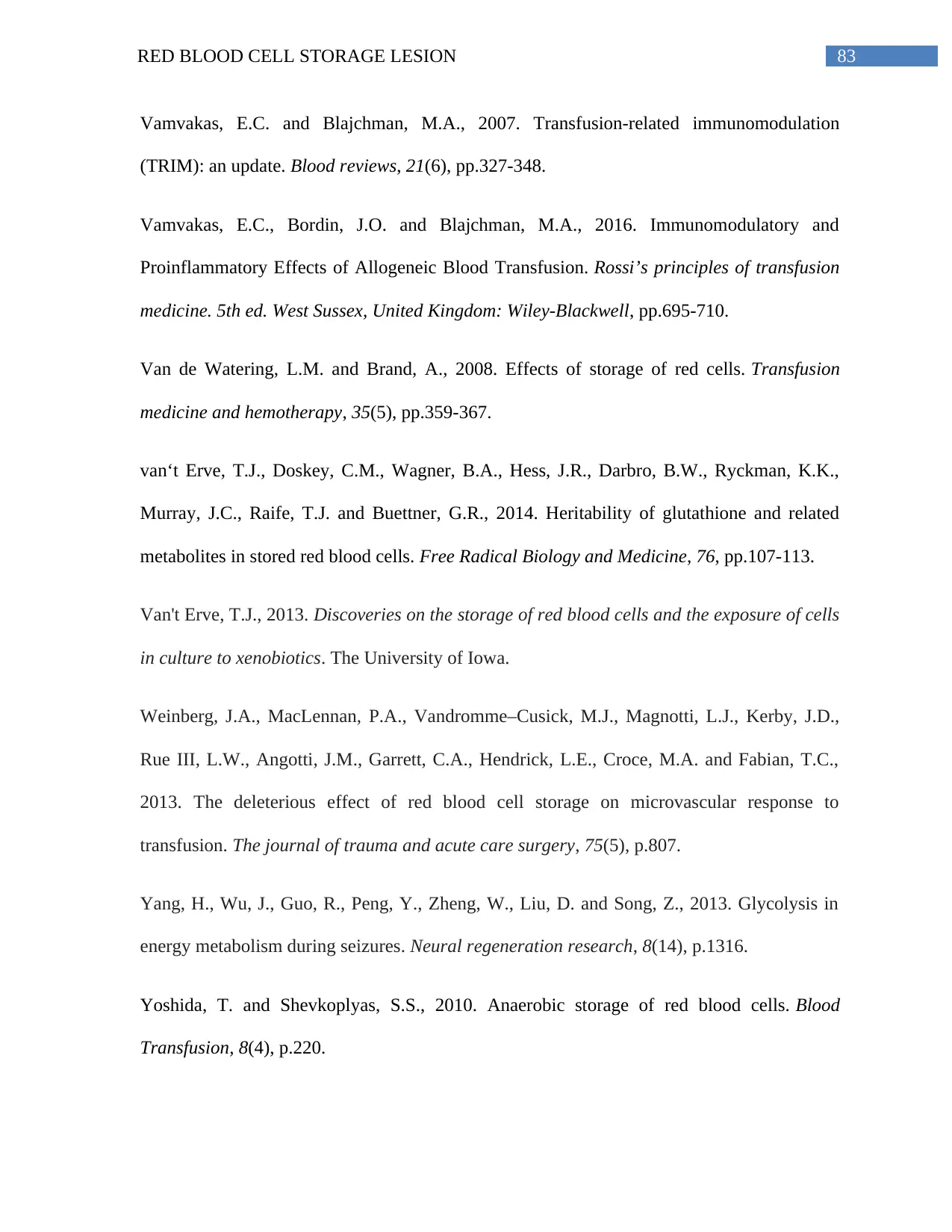
83RED BLOOD CELL STORAGE LESION
Vamvakas, E.C. and Blajchman, M.A., 2007. Transfusion-related immunomodulation
(TRIM): an update. Blood reviews, 21(6), pp.327-348.
Vamvakas, E.C., Bordin, J.O. and Blajchman, M.A., 2016. Immunomodulatory and
Proinflammatory Effects of Allogeneic Blood Transfusion. Rossi’s principles of transfusion
medicine. 5th ed. West Sussex, United Kingdom: Wiley-Blackwell, pp.695-710.
Van de Watering, L.M. and Brand, A., 2008. Effects of storage of red cells. Transfusion
medicine and hemotherapy, 35(5), pp.359-367.
van‘t Erve, T.J., Doskey, C.M., Wagner, B.A., Hess, J.R., Darbro, B.W., Ryckman, K.K.,
Murray, J.C., Raife, T.J. and Buettner, G.R., 2014. Heritability of glutathione and related
metabolites in stored red blood cells. Free Radical Biology and Medicine, 76, pp.107-113.
Van't Erve, T.J., 2013. Discoveries on the storage of red blood cells and the exposure of cells
in culture to xenobiotics. The University of Iowa.
Weinberg, J.A., MacLennan, P.A., Vandromme–Cusick, M.J., Magnotti, L.J., Kerby, J.D.,
Rue III, L.W., Angotti, J.M., Garrett, C.A., Hendrick, L.E., Croce, M.A. and Fabian, T.C.,
2013. The deleterious effect of red blood cell storage on microvascular response to
transfusion. The journal of trauma and acute care surgery, 75(5), p.807.
Yang, H., Wu, J., Guo, R., Peng, Y., Zheng, W., Liu, D. and Song, Z., 2013. Glycolysis in
energy metabolism during seizures. Neural regeneration research, 8(14), p.1316.
Yoshida, T. and Shevkoplyas, S.S., 2010. Anaerobic storage of red blood cells. Blood
Transfusion, 8(4), p.220.
Vamvakas, E.C. and Blajchman, M.A., 2007. Transfusion-related immunomodulation
(TRIM): an update. Blood reviews, 21(6), pp.327-348.
Vamvakas, E.C., Bordin, J.O. and Blajchman, M.A., 2016. Immunomodulatory and
Proinflammatory Effects of Allogeneic Blood Transfusion. Rossi’s principles of transfusion
medicine. 5th ed. West Sussex, United Kingdom: Wiley-Blackwell, pp.695-710.
Van de Watering, L.M. and Brand, A., 2008. Effects of storage of red cells. Transfusion
medicine and hemotherapy, 35(5), pp.359-367.
van‘t Erve, T.J., Doskey, C.M., Wagner, B.A., Hess, J.R., Darbro, B.W., Ryckman, K.K.,
Murray, J.C., Raife, T.J. and Buettner, G.R., 2014. Heritability of glutathione and related
metabolites in stored red blood cells. Free Radical Biology and Medicine, 76, pp.107-113.
Van't Erve, T.J., 2013. Discoveries on the storage of red blood cells and the exposure of cells
in culture to xenobiotics. The University of Iowa.
Weinberg, J.A., MacLennan, P.A., Vandromme–Cusick, M.J., Magnotti, L.J., Kerby, J.D.,
Rue III, L.W., Angotti, J.M., Garrett, C.A., Hendrick, L.E., Croce, M.A. and Fabian, T.C.,
2013. The deleterious effect of red blood cell storage on microvascular response to
transfusion. The journal of trauma and acute care surgery, 75(5), p.807.
Yang, H., Wu, J., Guo, R., Peng, Y., Zheng, W., Liu, D. and Song, Z., 2013. Glycolysis in
energy metabolism during seizures. Neural regeneration research, 8(14), p.1316.
Yoshida, T. and Shevkoplyas, S.S., 2010. Anaerobic storage of red blood cells. Blood
Transfusion, 8(4), p.220.
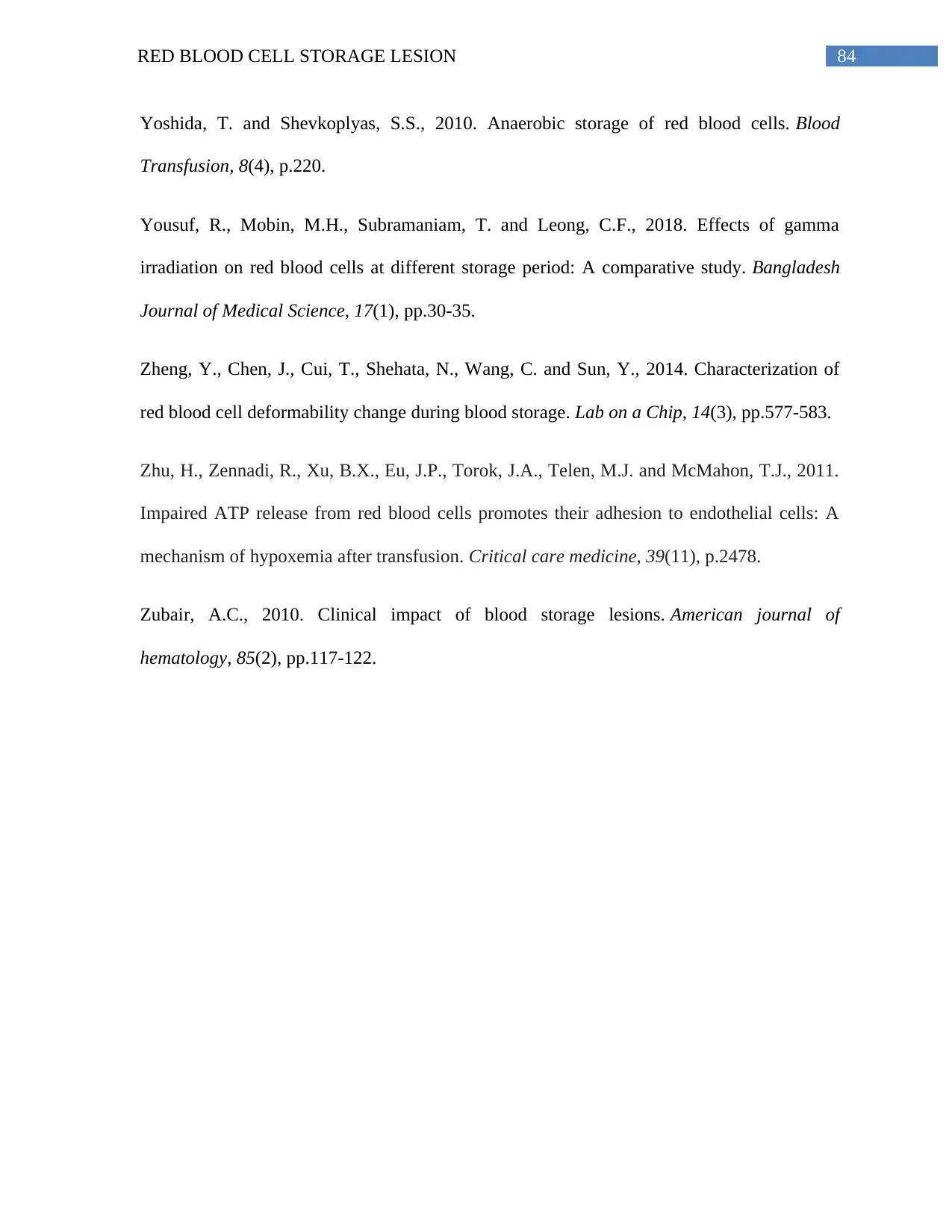
84RED BLOOD CELL STORAGE LESION
Yoshida, T. and Shevkoplyas, S.S., 2010. Anaerobic storage of red blood cells. Blood
Transfusion, 8(4), p.220.
Yousuf, R., Mobin, M.H., Subramaniam, T. and Leong, C.F., 2018. Effects of gamma
irradiation on red blood cells at different storage period: A comparative study. Bangladesh
Journal of Medical Science, 17(1), pp.30-35.
Zheng, Y., Chen, J., Cui, T., Shehata, N., Wang, C. and Sun, Y., 2014. Characterization of
red blood cell deformability change during blood storage. Lab on a Chip, 14(3), pp.577-583.
Zhu, H., Zennadi, R., Xu, B.X., Eu, J.P., Torok, J.A., Telen, M.J. and McMahon, T.J., 2011.
Impaired ATP release from red blood cells promotes their adhesion to endothelial cells: A
mechanism of hypoxemia after transfusion. Critical care medicine, 39(11), p.2478.
Zubair, A.C., 2010. Clinical impact of blood storage lesions. American journal of
hematology, 85(2), pp.117-122.
Yoshida, T. and Shevkoplyas, S.S., 2010. Anaerobic storage of red blood cells. Blood
Transfusion, 8(4), p.220.
Yousuf, R., Mobin, M.H., Subramaniam, T. and Leong, C.F., 2018. Effects of gamma
irradiation on red blood cells at different storage period: A comparative study. Bangladesh
Journal of Medical Science, 17(1), pp.30-35.
Zheng, Y., Chen, J., Cui, T., Shehata, N., Wang, C. and Sun, Y., 2014. Characterization of
red blood cell deformability change during blood storage. Lab on a Chip, 14(3), pp.577-583.
Zhu, H., Zennadi, R., Xu, B.X., Eu, J.P., Torok, J.A., Telen, M.J. and McMahon, T.J., 2011.
Impaired ATP release from red blood cells promotes their adhesion to endothelial cells: A
mechanism of hypoxemia after transfusion. Critical care medicine, 39(11), p.2478.
Zubair, A.C., 2010. Clinical impact of blood storage lesions. American journal of
hematology, 85(2), pp.117-122.
1 out of 85
![[object Object]](/_next/image/?url=%2F_next%2Fstatic%2Fmedia%2Flogo.6d15ce61.png&w=640&q=75)
Your All-in-One AI-Powered Toolkit for Academic Success.
+13062052269
info@desklib.com
Available 24*7 on WhatsApp / Email
Unlock your academic potential
© 2024 | Zucol Services PVT LTD | All rights reserved.